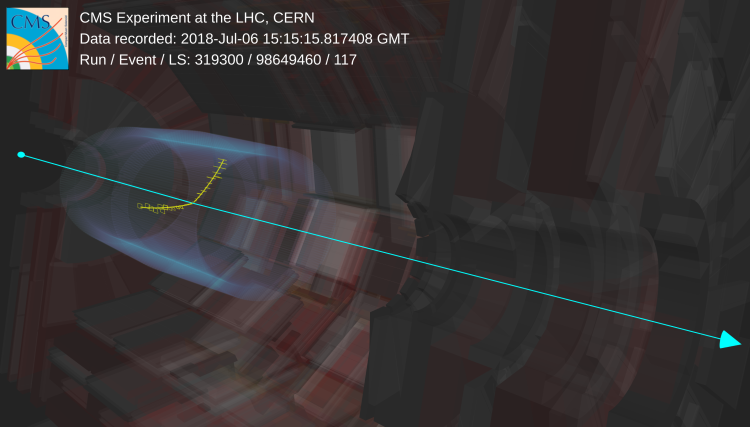
At the subatomic level, interactions between particles are mediated through the exchange of special particles, the force carriers. In the case of electromagnetism, the force is transmitted by photons. They connect particles with electric charge (electrons, protons, and many others). Photons are not charged, which is why the electromagnetic force has a long range and is relatively easy to calculate and test experimentally.
Another fundamental interaction of nature is the strong force. It acts between quarks through the exchange of gluons. The strong interaction holds the hadrons together, among them such important particles as protons or neutrons. In contrast to photons, gluons themselves carry colour charge, a quantum property that enables particles to interact via the strong force. This has two interesting consequences: the gluons change the colour of quarks in the interaction process and they can emit or absorb additional gluons. As a result, the strong interaction is indeed very strong at short scales, and rather difficult to master.
What happens when two very high-energy protons pass close to each other? They may scatter elastically at very small angles by exchanging a photon. Experiments revealed that there is another, more powerful scattering component. Where can that come from? The scattered protons (having zero net colour charge) remain intact, hence it cannot be the exchange of a single gluon! The simultaneous exchange of two (or more) gluons does the trick: interacting without getting coloured. In such cases the exchanged object is not an elementary particle but a collection of infinitely many “particle stories” (Feynman diagrams) involving gluons. Physicists call this object the pomeron, named after Isaak Pomeranchuk. The physics of elastic proton-proton scattering has been thoroughly studied by the TOTEM Collaboration at the LHC.
Figure 1: Illustration of non-resonant central exclusive production via double pomeron exchange. The pomerons emitted by the two protons are connected through a virtual hadron (h) and a particle-antiparticle pair is created (h+ and h-). An arbitrary number of additional exchanges between the two protons (shaded blob on the left) complicates the process.
We can also think of cases where the fusion of pomerons emitted by the two protons gives rise to new particles, such as the pair of hadrons shown in Fig. 1. These hadrons may be the decay products of a resonance or may appear through a non-resonant process involving a virtual hadron (called virtual because it has some of the characteristics of an ordinary particle but a different mass and is short-lived).
Such processes, called double-pomeron exchange, were jointly studied by the CMS and TOTEM Collaborations in a special data-taking campaign. The scattered protons are detected by two tracking detectors (“Roman pots”) installed in the LHC tunnel, about 200 m away from the nominal interaction point, each comprising ten silicon layers. They are moved very close to the LHC beam to detect even very small angle proton scatterings. The created particle-antiparticle pairs, mostly pions and kaons, are detected by the CMS silicon tracker, see Fig. 2.
Figure 2: Interactive display of a central exclusive event candidate as reconstructed by the CMS and TOTEM experiments. The directions of the two outgoing scattered protons are marked by light blue arrows. The yellow trajectories illustrate the two centrally produced charged pions, curved in the magnetic field. The little rectangles indicate silicon detector units with hit clusters. Try to zoom and rotate in this separate page.
For each collision event, we measure the momentum components and charge of all four particles: the two forward-going protons and the centrally-produced hadrons. The background is suppressed by requiring momentum conservation. Non-resonant processes were studied to precisely measure several parameters of the pomeron, such as the strengths of the coupling of the proton to the pomeron and of the pomeron to the hadrons, and their energy dependence. These quantities are measured through a lengthy but automatised theoretical model-tuning study.
Figure 3: Differential cross section as a function of 𝜙 for several transverse momentum ranges of the scattered protons. The measured values (markers) are compared to the predictions of several theoretical models (bands) using the tuned parameters. The black curves show the results of fits with the function [A(R – cos 𝜙)].
Figure 3 shows the distributions of the azimuthal angle 𝜙 between the two out-going protons, for several ranges in their transverse momentum. It is the first time that such measurements have been made with such a high precision. Despite the complexity of the underlying physics, the distributions have a fairly simple cosine shape that changes with transverse momenta. The parabolic minimum, corresponding to an almost zero cross section, results from a destructive interference at the quantum level, happening when two or more competing microscopical processes contribute to the final outcome of a collision. This detailed measurement of pomeron-related quantities has given us a close insight into how the strong interaction works at these energies.
What next? With this new knowledge about pomerons, we will turn our attention to the produced resonances. Will we only observe the well-known scalar and tensor mesons, or will we see something unexpected? Will we see signs of a quarkless particle, the glueball, in such a gluon-rich environment? The ongoing Run 3 of the LHC and future data taking campaigns, with the help of the Precision Proton Spectrometer of CMS, will provide us with much more data to tackle these exciting questions.
Read more about these results:
-
CMS Physics Analysis Summary (SMP-21-004): "Nonresonant central exclusive production of charged hadron pairs in proton-proton collisions at 13 TeV"
-
Display of collision events: CERN CDS
-
@CMSExperiment on social media: LinkedIn - facebook - twitter - instagram
- Do you like these briefings and want to get an email notification when there is a new one? Subscribe here