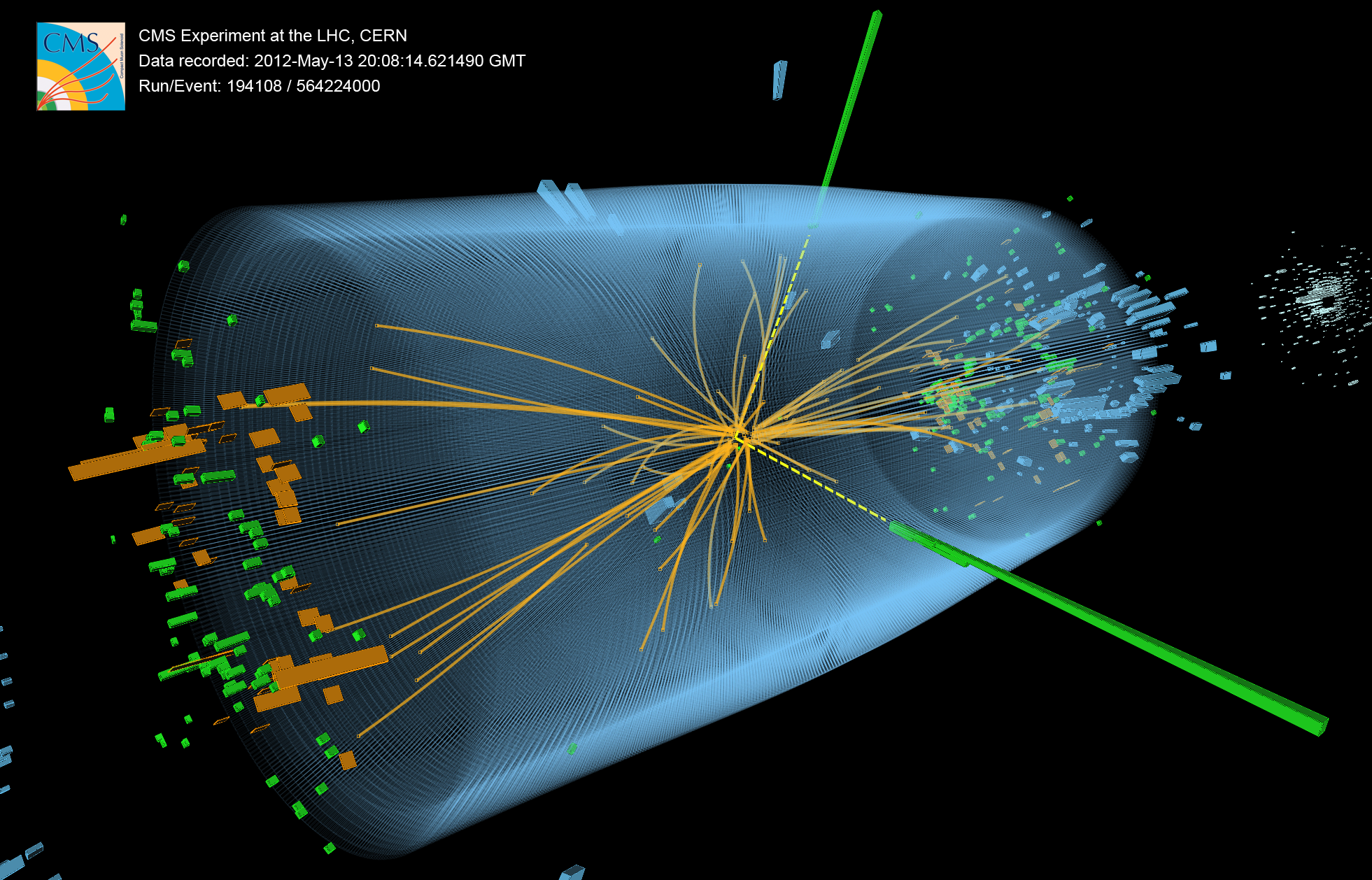
Following the results presented at the electroweak session of the annual winter conference, Rencontres de Moriond, CMS has now presented many new results for this week’s quantum chromodynamics (QCD) session. The results include many searches for new physics as well as some of the best measurements to date of known Standard Model processes. A few of the results are highlighted below.
The Higgs hunt
Decays to two photons: HIG-13-001
CMS has performed a search for the Standard Model Higgs boson in many different channels corresponding to how the boson would decay. One of these is the two-photon or γγ channel. Since July 2012, CMS has more than doubled the data sample. Experts also improved the analysis methods and the energy calibration of the electromagnetic calorimeter, the CMS subdetector that measures photons. Using these improvements, CMS performed two different analyses on the full dataset of ~5 fb−1 (inverse femtobarn[1]) and ~20 fb−1 of proton-collision data collected at a centre-of-mass energy of 7 and 8 TeV[2] respectively. The two analyses, which use independent techniques applied to the same data samples, are statistically consistent and show that the excess of events at around 125 GeV remains, with a significance of 3.2σ and 3.9σ[3] respectively. The rate of production with subsequent decay into two photons divided by the expected rate for a SM Higgs gives a ratio (μ) of around 0.8 ± 0.3 for the first analysis which is the official CMS preliminary result and 1.1 ± 0.3 for the second which is the cross-check analysis, both of which are consistent with the expectation for a SM Higgs boson.
Decays to two τ (tau) leptons: HIG-13-004
CMS has also released additional results from the Higgs search in the τ channel, in which we see a strong indication of a signal (see Moriond EW statement). HIG-13-004 Figure 1 shows the likelihood of the signal as a function of the visible mass of the ττ system, which, if there is a signal present in the data, should produce a minimum value at mass of the particle producing the two τs. We find a best-fit value for the Higgs mass in this channel of 120 ± 9 GeV, consistent with the precision measurements in the two-photon and ZZ channels.
Searches for exotic physics
Dark-matter candidates and more: EXO-12-048
Many models of new physics — those with dark-matter candidates, extra dimensions or an entirely new class of particles known as “Unparticles” — are predicted to manifest in events with a single jet and large missing energy. Particles such as neutrinos cannot be detected by CMS and their presence has to be inferred from missing energy in a collision event. Particles such as dark-matter candidates or Unparticles will also not be directly detected by CMS and will leave behind clear signatures of a lot of missing energy (as shown in EXO-12-048 Figure 1). CMS looked through the full 20 fb−1 of 8TeV proton-collision data and, in the absence of any significant deviations from the background expectations, has set limits on these models of new physics. For example, the search pushes the lower limits of the fundamental scale MD to beyond 5 TeV for two extra dimensions. Within the framework of a simple model for the production of dark matter, the analysis is particularly sensitive in the low-mass region below 3.5 GeV if the spin of the produced particles is ignored, and it can set the world’s best limits in the spin-dependent case.
Looking for heavier quarks: B2G-12-012
Several theories, which allow the Standard Model to function without the fine-tuning of its parameters, propose the existence of partners for the top quark. One such partner is the T5/3, a heavy quark with 5/3rd the charge of an electron. This heavy quark is predicted to decay into a top quark and a W boson. CMS has looked for this top partner in the entire 2012 dataset (~20 fb−1 at 8 TeV centre-of-mass energy) by considering events where the decay of this exotic quark results in a pair of leptons (electrons or muons) with the same electric charge. No significant excess of such events was observed, and CMS excludes the existence of the T5/3 top partners with masses below 770 GeV at the 95% confidence level[4]. This is the most stringent limit on the existence of such top partners to date. [Note: This result was presented at Moriond EW. Our apologies about the omission in our previous statement.]
The search for heavy gauge bosons: B2G-12-010
We know of three types of gauge bosons: the photon, the Z boson and the W boson (which can be W+ or W−). Various extensions of the Standard Model predict the existence of much heavier bosons, such as the W′ (called W-prime). One of the ways in which the W′ boson can decay is into top and bottom quarks (W′→tb). This channel is important because in many models the third generation (top and bottom quarks) is expected to be coupled more strongly to the W′ than the first (up and down quarks) and second generations (charm and strange quarks). This search uses the entire 2012 dataset to analyse events with a lepton (electron, muon) as well as particle jets and missing transverse energy. CMS finds no significant excess in the data and rules out W′ bosons with masses up to 2.03 TeV. These limits represent a big improvement over previously published results.
Standard Model measurements
Despite requiring its parameters to be extremely fine-tuned, the Standard Model of particle physics has been incredibly robust for decades. Standard Model processes contribute to the background of many searches for new physics and measuring these processes precisely helps separate the background from a potential signal. In addition, any deviation from the Standard Model’s predictions could be the door that leads to new physics.
Do matter and anti-matter have the same mass?: TOP-12-031
Fundamental symmetries of the Standard Model tell us that particles and their anti-particles should have the same mass. The top quark, the heaviest particle ever observed, makes an excellent candidate to test if these symmetries hold. CMS went through around 300,000 top-antitop pairs decaying in a variety of ways in nearly 20 fb−1 of 8TeV data to calculate the mass difference, represented as Δmt = mt − manti-t. This value was found to be −272 ± 196 (stat.) ± 122 (syst.) MeV, well in agreement with the Standard Model expectations. (The central value is around 0.15% of the mass of the top quark, which is ~173 GeV or ~173,000 MeV.)
Production of W bosons in association with quarks: SMP-12-002, SMP-12-026
CMS has performed a first-of-its-kind measurement, studying the rate of production of a W boson associated with a charm quark, and also calculating, for this production mode, the ratio W+ bosons to W− ones. The W bosons are produced thus: gluons inside the colliding protons in the LHC can split into quark-antiquark pairs; if the gluon splits into a charm-anticharm pair (as shown in SMP-12-002 Figure 1), the anti-charm quark may combine with a strange quark from another proton to form a W boson (a W− in this case while a W+ is produced from the fusion of a charm quark and an anti-strange quark). The W boson then decays into a lepton (an electron or a muon) and an undetected neutrino, while the remaining charm quark produces particle jets, that can be detected by CMS. CMS performed this measurement by going through 5 fb–1 of 7TeV data collected in 2011, looking for events containing a jet from the charm quark (c-jet) as well as decays of the W into a lepton and a neutrino. This is the first time that a direct access to the strangeness component of the proton has been performed at the LHC. A related measurement involves the production of a W boson along with a bottom-antibottom pair. In 5 fb−1 of 7TeV data, CMS studied events with two b-jets and a W decaying into a muon and a neutrino, and found the production rates to agree with the theoretical predictions.
More information
- This statement is available online at: http://cmsexperiment.web.cern.ch/news/new-cms-results-moriond-qcd-2013
- CMS public website: http://cern.ch/cms
- CERN Press Release, 1 March 2012
References
- Simple explanations of Higgs search terminology
- Explanation of the Higgs boson exclusion plots (on atlas.ch)
- Blinding and unblinding analyses
CMS Physics
- All CMS Papers
- All CMS Physics Analysis Summaries
- All CMS results
- Images of real collisions in CMS
- Animations of real collisions in CMS
- Receive updates on latest CMS papers and more | Follow @CMSpapers on Twitter
About CMS
More information: http://cern.ch/cms, or contact: cms.outreach@cern.ch.
CMS is one of two general-purpose experiments at the LHC that have been built to search for new physics. It is designed to detect a wide range of particles and phenomena produced in the LHC's high-energy proton-proton and heavy-ion collisions and will help to answer questions such as: "What is the Universe really made of and what forces act within it?" and "What gives everything mass?" It will also measure the properties of well-known particles with unprecedented precision and be on the lookout for completely new, unpredicted phenomena. Such research not only increases our understanding of the way the Universe works, but may eventually spark new technologies that change the world in which we live as has often been true in the past.
The conceptual design of the CMS experiment dates back to 1992. The construction of the gigantic detector (15 m diameter by nearly 29 m long with a weight of 14000 tonnes) took 16 years of effort from one of the largest international scientific collaborations ever assembled: 3275 physicists (including 1535 students) plus 790 engineers and technicians, from 179 institutions and research laboratories distributed in 41 countries all over the world.
Footnotes
[1] http://news.stanford.edu/news/2004/july21/femtobarn-721.html
[2] The electron volt (eV) is a unit of energy. A GeV is 1,000,000,000 eV; a TeV is 1000 GeV. In particle physics, where mass and energy are often interchanged, it is common to use eV/c2 as a unit of mass (from E = mc2, where c is the speed of light in vacuum). Even more common is to use a system of natural units with c set to 1 (hence, E = m), and use eV and GeV as units of mass.
[3] The standard deviation describes the spread of a set of measurements around the mean value. It can be used to quantify the level of disagreement of a set of data from a given hypothesis. Physicists express standard deviations in units called “sigma”. The higher the number of sigma, the more incompatible the data are with the hypothesis. Typically, the more unexpected a discovery is, the greater the number of sigma physicists will require to be convinced.
[4] Confidence level is a statistical measure of the percentage of test results that can be expected to be within a specified range. For example, a confidence level of 95% means that the result of an action will probably meet expectations 95% of the time.
- Log in to post comments