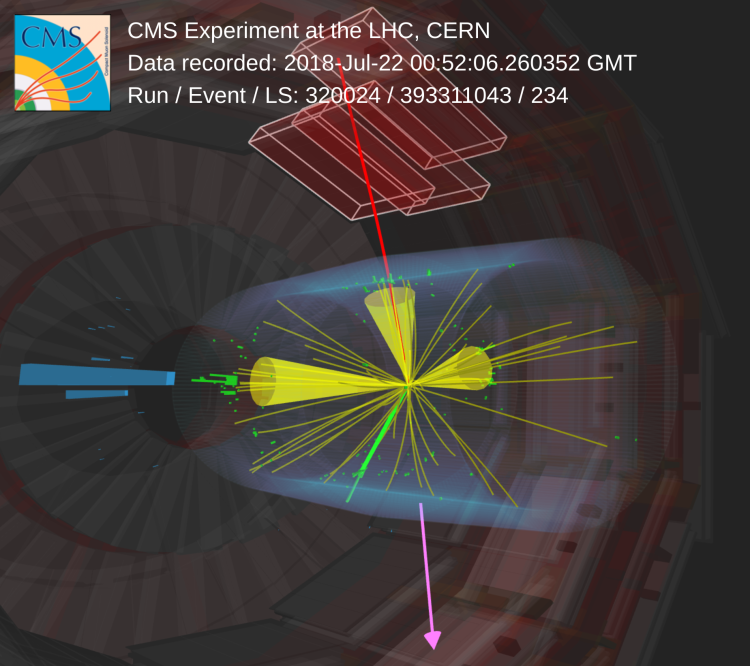
Peter Higgs and François Englert received the Nobel Prize in Physics after the 2012 discovery of the Higgs boson with a mass of 125 GeV. Its existence provided experimental evidence of the predicted ‘Higgs field’ that pervades the universe and provides the mechanism that gives mass to the fundamental building blocks of nature. How does this impact the real world? Consider the simplest atom, hydrogen, which consists of a proton and an electron. If electrons were massless, they would not bind to the nucleus! In such a scenario, the electromagnetic attraction between protons and electrons would not be sufficient to hold them together, meaning we wouldn't have atoms, molecules, plants, planets or stars. The entire universe would look very different.
Interestingly, there are no limitations in the standard model (SM) of particle physics that prevent the existence of more than one ‘Higgs boson’. In fact, many sophisticated theories go beyond the SM (BSM) and predict that the Higgs boson has several siblings, the existence of which could help us understand several unexplained physics phenomena, such as the matter-antimatter imbalance in the universe, the production of dark matter, or the heaviness of the top quark and the SM Higgs boson compared to other known particles.
The ‘two-Higgs-doublet’ model (2HDM) is a theory that predicts four siblings for the Higgs boson, denoted as A, H, H+, and H-. The first two are electrically neutral and the last two have positive and negative electric charges, respectively. The masses of the H and A bosons can be below 1 TeV, meaning that if they exist, we might find them in the LHC proton-proton collisions! So far, this possibility has not been ruled out.
Figure 1. Illustration of the top-quark-associated production of a new boson, H or A, that decays to a top quark and a charm (or up) antiquark.
The CMS Collaboration recently released a novel search for the neutral bosons, H or A, produced in association with a top quark. This is an interesting search channel as the interaction strength between the neutral siblings of the Higgs boson and other fundamental particles is stronger for particles with larger masses. The top quark is the heaviest particle we know to date, meaning that the process we chose would have a very large probability to occur. Figure 1 schematically illustrates the production of the new neutral bosons: the blue line represents H or A, and the downward green line represents a simultaneously produced top quark. The neutral boson subsequently decays into a charm (or up) antiquark and a top quark. In this sense, the behavior of the H and/or A boson is different from the discovered Higgs boson of SM: the SM Higgs boson cannot decay to a quark and antiquark that have different flavors.
As is the case 99% of the time, each of the two top quarks in our signal is expected to decay into a b quark and a W boson. This search targets events where both W bosons decay into a charged lepton and a neutrino. The final state we observe with the CMS detector contains two leptons of the same electric charge, together with two b-quark jets and one up or charm antiquark jet. Though such events are rare, this selection significantly reduces background contributions. Sophisticated machine learning techniques are optimized to enhance the sensitivity of the analysis to this signal against SM processes featuring a similar signature.
Figure 2: A proton-proton collision event at a center-of-mass energy of 13 TeV, recorded by the CMS detector. The signature is similar to what is expected from a top-quark-associated A/H production where both top quarks eventually decay to leptons. It is characterized by presence of two leptons (here a muon and an electron) with the same electric charge, two b jets from the decay of the top quarks, and a third jet from the A/H decay. The muon is represented by the red line, the electron by the green line, and jets by the yellow cones. Try to rotate and zoom on this separate page.
The analysis used the data collected by CMS during 2016–2018, corresponding to an integrated luminosity of 138 fb-1. As the mass of the new neutral bosons is not known, we performed the search for many scenarios where the mass of H (or A) can vary from 200 GeV to 1 TeV. No statistically significant excess over the SM backgrounds is observed. Figure 3 presents the observed upper limits on the ratio between the measured signal production cross section and that expected from the theory. The results are presented as a function of the mass of the neutral boson A, and the strength of its interaction with the particles it decays to: a top quark and charm antiquark, or a top antiquark and a charm quark.
Figure 3. Observed upper limits for the ratio between the measured signal production cross section and that expected from the theory, as a function of the mass of the neutral boson A, and the strength of its interaction with the top quark and charm antiquark, or with the top antiquark and charm quark.
Regions where the upper limit of this ratio is below one are ruled out, as we can be confident that what we observe is lower than what we would expect, on the basis of this theory. Despite the lack of a significant excess, these are some of the most informative results that we have achieved for this model until now and provide physicists with valuable insights towards future directions of exotic Higgs boson searches. The wealth of data that will be collected over the next decade at the LHC, along with continuously-improving experimental methods, will certainly enhance the sensitivity of searches for the ‘new physics’ that we know must be out there. New Higgs bosons or something else, physics is currently at a crossroads and what we will find in the next few years will have a monumental impact on the path it takes.
Read more about these results:
-
CMS Physics Analysis Summary (TOP-22-010): "Search for new Higgs bosons through same-sign top quark pair production in association with a jet in proton-proton collisions at 13 TeV"
-
@CMSExperiment on social media: LinkedIn - facebook - twitter - instagram
- Do you like these briefings and want to get an email notification when there is a new one? Subscribe here