Physics
PhysicsCMS Scientific Results
CMS Scientific ResultsThe CMS Collaboration has a broad physics programme, ranging from measurements of Standard Model quantities and the recently discovered Higgs boson, to studies of heavy-ion collisions, and searches for new particles, phenomena, and even extra dimensions in the Universe. The collaboration has published over 1000 scientific papers since 2010. CMS results from LHC collision data collected with the CMS detector are made public via papers (published or submitted for publication) and public notes (PAS) describing preliminary results. CMS also releases public notes that include detector and performance plots (DP), results on physics upgrade studies as well as Technical Design Reports. Many of these results also provide an article targeting the general public which can be found on this page.
Summary plots by physics topic: SMP, TOP, NPS, EXO, B2G, HIG
The CMS Physics Coordination area has 8 groups dedicated to different thematic physics analyses (PAGs), and 8 groups focused on physics objects (POGs). There are 4 groups (SG) in charge of reconstruction, simulation, and algorithms and 2 cross-coordinated groups among PAGs and POGs. The Physics Coordination office provides support in the development of triggers and in the development, maintenance, and use of analysis data formats (XPOG). The PC communication office prepares content for the dissemination of CMS physics results and sustains communication with the wider HEP community.
Physics Coordination Office |
||
Cross-POG (XPOG) Officers (email): Huilin Qu (CERN), Felipe Silva (RIO)
|
Physics Communications Officers (email): Ansar Iqbal (UCLA), Andres Delannoy (TENNESSEE) |
Trigger Officer (email): Artur Lobanov (HAMBURG), Aruna Nayak (NISER) |
Physics Object Groups (POGs) |
BTV: B-tagging and Vertexing Conveners (email): S. Mondal (BROWN), S. Wuchterl (CERN) Public page |
TRK: Tracking Conveners (email): M. Masciovecchio (UCSD), K. Skopven (GHENT) Public page |
EGM: Electron and Photons Conveners (email): R. Tramontano (ZURICH), R. Salvatico (CERN) Public page |
JME: Jet and Missing Energy Conveners (email): M. Cremonesi (CARNEGIE-MELLON), A. Benecke (LOUVAIN) Public page |
MUO: Muons Conveners (email): M. Bonanomi (HAMBURG), F. Primavera (BOLOGNA) Public page |
TAU: Taus Conveners (email): M. Bluj (WARSAW-INS), A. Cardini (DESY) Public page |
LUM: Luminosity Conveners (email): J.Benitez (SONORA), F. Romeo (VANDERBILT) Public page |
PRO: Protons (in PPS) Conveners (email): L.Forthomme (KRAKOW), A. Bellora (TORINO) Public page |
Physics Analysis Groups (PAGs) |
BPH: B Physics and Quarkonia Conveners (email): G. Karathanasis (CERN), D. Kovalskyi (MIT) Public page |
SMP: Standard Model Physics Conveners (email): Y. Maravin (KANSAS-STATE), M. Pelliccioni (TORINO) Public page |
TOP: Top Physics Conveners (email): S. Sanchez Cruz (CERN), A. Grohsjean (HAMBURG) Public page |
HIG: Higgs Physics Conveners (email): M. Xiao (ZHEJIANG-UNIV), E. Di Marco (ROMA) Public page |
NPS: New Physics searches with Standard objects Conveners (email): S. Sekmen (KYUNGPOOK), C. Caillol (CERN) Public page |
EXO: Searches for Exotica Conveners (email): B. Maier (LONDON-IC), C. Pena (FNAL) Public page |
B2G: Searches for Beyond SM particles decaying to top quarks and Higgs and Gauge bosons Conveners (email): L. Gouskos (BROWN), A. Reimers (UZH) Public page |
HIN: Heavy-Ion Physics Conveners (email): L. Cunqueiro Mendez (ROMA-1), J. Wang (CERN) Public page |
Shared Groups |
|||
Generator and MC production shared with O&C Conveners (email): L.Viliani (FIRENZE), B. Bilin (CERN) Public page |
Machine Learning shared with O&C Conveners (email): P.Vischia (OVIEDO), J. Duarte (UCSD) Public page |
Particle Flow shared with PPD Conveners (email): S. Mukherjee (AACHEN), R. Bellan (TORINO) Public page |
Cross-group activities |
|||
EFT Forum Conveners (email): G. Boldrini (MILANO BICOCCA), Matteo Presilla (KIT), A. Calandri (ETH), J. Wilson (BAYLOR) |
PDF Forum Conveners (email): K. Wichmann (DESY), K. Rabbertz (KIT) |
Monte Carlo & Interpretation Forum Conveners (email): T. Almos Vami (UCSB), S. Qian (PEKING), R. Wolf (KARLSRUHE), A. Agapitos (PEKING), J. Choi (SEOUL), S. Fiorendi (TENNESSEE), V. Hegde (TEXAS-TECH), M. Lu (NORTHEASTERN), A. Carvalho (PEKING) |
Common Analysis Tools Conveners (email): A.Nigamova (HAMBURG), P. Lenzi (FLORENCE) |
Glossary
GlossaryA | B | C | D | E | F | G | H | I | J | K | L | M | N | O | P | Q | R | S | T | U | V | W | X | Y | Z
Accelerating cavity
Accelerating cavities produce the electric field that accelerates the particles inside particle accelerators. Because the electric field oscillates at radio frequency, these cavities are also referred to as radio-frequency cavities.
Accelerator
A machine in which beams of charged particles are accelerated to high energies. Electric fields are used to accelerate the particles while magnets steer and focus them. Beams can be made to collide with a static target or with each other.
- A collider is a special type of circular accelerator where beams travelling in opposite directions are accelerated and made to interact at designated collision points.
- A linear accelerator (or linac) is often used as the first stage in an accelerator chain.
- A synchrotron is an accelerator in which the magnetic field bending the orbits of the particles increases with the energy of the particles. This makes the particles move in a circular path.
AD
The Antiproton Decelerator, the CERN research facility that produces the low-energy antiprotons.
ALICE (A Large Ion Collider Experiment)
One of the four large experiments that will study the collisions at the LHC.
Antimatter
Every kind of matter particle has a corresponding antiparticle. Charged antiparticles have the opposite electric charge to their matter counterparts. Although antiparticles are extremely rare in the Universe today, matter and antimatter are believed to have been created in equal amounts at the Big Bang.
Antiproton
The antiparticle of the proton.
ATLAS
One of the four large experiments that will study the collisions at the LHC.
Atom
All ordinary matter is made up of atoms, which are themselves composed of a nucleus and electrons. The protons and neutrons in the nucleus are made of quarks, the smallest known matter particles.
Beam
The particles in an accelerator are grouped together in a beam. Beams can contain billions of particles and can be divided into discrete portions called bunches. Each bunch is typically several centimetres long and just a few microns wide.
Big Bang
The name given to the explosive origin of the Universe.
Boson
The collective name given to the particles that have integer (whole number) spin and carry forces between particles of matter. (See also Particles.)
Calorimeter
An instrument for measuring the amount of energy carried by a particle. In particular, the electromagnetic calorimeter measures the energy of electrons and photons, whereas the hadronic calorimeter determines the energy of hadrons, that is, particles such as protons, neutrons, pions and kaons.
CARE (Co-ordinated Accelerator Research in Europe)
An EU-supported activity to generate a structured and integrated area in accelerator research and development in Europe.
Cherenkov radiation
Light emitted by fast-moving charged particles traversing a dense transparent medium faster than the speed of light in that medium.
CLIC (Compact LInear Collider)
A site-independent feasibility study aiming at the development of a realistic technology at an affordable cost for an electron–positron linear collider for physics at multi-TeV energies.
CMS (Compact Muon Solenoid)
One of the four large experiments that will study the collisions at the LHC.
CNGS (CERN Neutrinos to Gran Sasso)
A project that aims at the first observation of the tau neutrino by sending a beam of muon neutrinos from CERN to the Laboratori Nazionali del Gran Sasso in Italy.
Collider
Special type of acclerator where counter-rotating beams are accelerated and interact at designated collision points. The collision energy is twice that of an individual beam, which allows higher energies to be reached than in fixed target accelerators.
Colour charge
Colour charge is a property of quarks and gluons analogous to electric charge. It is merely a term relating to the strong forces of interaction, and has nothing to do with the visual notion of colour. The three "colours" a quark may take are red, blue or green; those an anti-quark may take are anti-red, anti-blue or anti-green; while a gluon has a colour-anticolour combination.
Cosmic ray
A high-energy particle that strikes the Earth’s atmosphere from space, producing many secondary particles, also called cosmic rays.
CP violation
A subtle effect observed in the decays of certain particles that betrays Nature’s preference for matter over antimatter.
Cross section
A quantity proportional to the probability for a specified reaction (such as creation of a new particle) to occur, for example when the two proton beams collide as in the LHC. The name reflects the origin of the concept in classical mechanics (geometrical cross-sectional area of an object which could be hit by a beam), but in particle physics the probabilities are from quantum mechanics. At the LHC, cross sections are typically expressed in nanobarns (nb), picobarns (pb), and femtobarns (fb).
Cryogenic distribution line (QRL)
The system used to transport liquid helium around the LHC at very low temperatures. This is necessary to maintain the superconducting state of the magnets that guide the particle beam.
Cryostat
A refrigerator used to maintain extremely low temperatures.
CMSSM
The constrained minimal supersymmetric extension of the standard model, CMSSM, is a simplified and practical model of SUSY-breaking that is often used to illustrate and compare the reach of SUSY searches at colliders.
Dark matter
Only 4% of the matter in the Universe is visible. The rest is known as dark matter — 26%, and dark energy — 70%. Finding out what it consists of is a major challenge for modern science.
Detector
A device used to measure properties of particles. Some detectors measure the tracks left behind by particles, others measure energy. The term ‘detector’ is also used to describe the huge composite devices made up of many smaller detector elements. In the large detectors at the LHC each layer has a very specific task.
Dipole
A magnet with two poles, like the north and south poles of a horseshoe magnet. Dipoles are used in particle accelerators to keep particles moving in a circular orbit. In the LHC there are 1232 dipoles, each 15 m long.
Enabling Grids for E-SciencE (EGEE) project
An EU-funded project led by CERN, now involving more than 90 institutions in over 30 countries worldwide, to provide a seamless Grid infrastructure that is available to scientists 24 hours a day.
Electronvolt (eV)
A unit of energy or mass used in particle physics. One eV is extremely small, and units of a million electronvolts, MeV, or thousand million electronvolts, GeV, are more common. The latest generation of particle accelerators reaches up to several million million electronvolts, TeV. One TeV is about the energy of motion of a flying mosquito.
Electromagnetic force
The electromagnetic force binds negative electrons to the positive nuclei in atoms, and underlies the interactions between atoms that give rise to molecules and to solids and liquids. Unlike gravity, it can produce both attractive and repulsive effects. Opposite electric charges (positive and negative) and opposite magnetic poles (north and south) attract, but charges or poles of the same type repel each other.
Electroweak interactions
The name given to the interactions (electromagnetic and weak) which were unified in the theory for which Glashow, Salam, and Weinberg received the Nobel Prize in 1979. The electroweak force carriers are the positively and negatively charged W bosons, and the electrically neutral photon (γ) and Z boson.
End-cap
Detector placed at each end of a barrel-shaped detector to provide the most complete coverage in detecting particles.
Exclusions
If searches for a particle reveal statistically that it almost certainly doesn’t exist with certain characteristics (e.g. a particular mass), future searches exclude those characteristics. This narrows the search parameters within which the particle might be found. Establishing such exclusions is very important in the search for undiscovered particles.
Forces
There are four known fundamental forces in nature. Gravity is the most familiar to us, but it is the weakest. Electromagnetism is the force responsible for lightning and carrying electricity into our homes. The two other forces, weak and strong, are confined to the atomic nucleus. The strong force binds the nucleus together, whereas the weak force causes some nuclei to break up. The weak force is important in the energy-generating processes of stars, including the Sun. Physicists would like to find a theory that can explain all these forces. A big step forward was made in the 1960s when the electroweak theory uniting the electromagnetic and weak forces was proposed. This was later confirmed in a Nobel-prize-winning experiment at CERN.
Fundamental particle
One of the smallest known particles, from which all the other particles are made of.
Gluon
Gluon is a special particle, called boson, that carries the strong force, one of the four fundamental forces, or interactions, between particles.
Hadron
A subatomic particle that contains quarks, antiquarks, and gluons, and so experiences the strong force. (See also Particles.)
Higgs boson
A particle predicted by theory. It is linked with the mechanism by which physicists think particles acquire mass.
Injector
System that supplies particles to an accelerator. The injector complex for the LHC consists of several accelerators acting in succession.
Ion
An ion is an atom with one or more electrons removed (positive ion) or added (negative ion).
Isotope
Slightly different versions of the same element, differing only in the number of neutrons in the atomic nucleus—the number of protons is the same.
Kaon
A meson containing a strange quark (or antiquark). Neutral kaons come in two kinds, long-lived and short-lived. The long-lived ones occasionally decay into two pions, a CP-violating process. (See also Particles.)
Kelvin
A unit of temperature. One kelvin is equal to one degree Celsius. The Kelvin scale begins at absolute zero, –273.15°C, the coldest temperature possible.
LCG (LHC Computing Grid)
The mission of the LCG is to build and maintain a data-storage and analysis infrastructure for the entire high-energy physics community that will use the LHC.
LEP
The Large Electron–Positron Collider, which ran at CERN until 2000.
Lepton
A class of elementary particle that includes the electron. Leptons are particles of matter that do not feel the strong force. (See also Particles.)
LHC
The Large Hadron Collider, CERN’s biggest accelerator.
LHCb (Large Hadron Collider beauty)
One of the four large experiments that will study the collisions at the LHC.
Linac
An abbreviation for linear accelerator.
Mass, invariant mass, effective mass
Particle physicists use the word "mass" to refer to the quantity (sometimes called "rest mass") which is proportional to the inertia of the particle when it is at rest. This is the "m" both in Newton's second law of motion, F=ma, and in Einstein's equation, E=mc2 (in which E must be interpreted as the energy of the particle at rest). When a particle decays and hence no longer exists, its mass before the decay can be calculated from the energies and momenta of the decay products. The inferred value of the mass is independent of the reference frame in which the energies and momenta are measured, so that that the mass called "invariant". The concept is frequently generalized, so that for any set of particles (e.g., two leptons emerging from a collision), one can apply the same formulas to obtain an "invariant mass" (also called the “effective mass”) of the set.
Mass spectrum
One of the important statistical tools in particle physics is to create a histogram of the invariant mass of a particle or a group of particles (thought to originate from the decay of something interesting) versus the frequency with which that particular mass was recorded. This plot is known as a mass spectrum, and is used to signify the presence of new particles and to establish their masses.
Model / Scientific model
A model is a representation of phenomena or processes that allows us to summarise and study them. They can be made up of a number of hypotheses working within a set of parameters, usually obtained from observation and/or experiment. Modelling, including the use of computer simulations, is an essential part of scientific activity as it gives us a framework within which to test our hypotheses - we can create a complex model, alter its parameters, test its reliability against the real world and even make predictions.
Muon
A particle similar to the electron, but some 200 times more massive. (See also Particles.)
Muon chamber
A device that identifies muons, and together with a magnetic system creates a muon spectrometer to measure momenta.
Neutrino
A neutral particle that hardly interacts at all. Neutrinos are very common and could hold the answers to many questions in physics. (See also Particles.)
Neutron
A baryon with electric charge zero; it is a hadron with a basic structure of two down quarks and one up quark (held together by gluons).
Nucleon
The collective name for protons and neutrons.
Particles
There are two groups of elementary particles, quarks and leptons. The quarks are up and down, charm and strange, top and bottom. The leptons are electron and electron neutrino, muon and muon neutrino, tau and tau neutrino. There are four fundamental forces, or interactions, between particles, which are carried by special particles called bosons. Electromagnetism is carried by the photon, the weak force by the charged W and neutral Z bosons, the strong force by the gluon; gravity is probably carried by the graviton, which has not yet been discovered. Hadrons are particles that feel the strong force. They include mesons, which are composite particles made up of a quark–antiquark pair and baryons, which are particles containing three quarks. Pions and kaons are types of meson. Neutrons and protons (the constituents of ordinary matter) are baryons; neutrons contain one up and two down quarks; protons two up and one down quark.
Photon
The force carrier particle of electromagnetic interactions.
See Particles.
Pion
The least massive type of meson.
Positron
The antiparticle of the electron.
Proton
The most common hadron, a baryon with electric charge +1 equal and opposite to that of the electron. Protons have a basic structure of two up quarks and one down quark (bound together by gluons). The nucleus of a hydrogen atom is a proton.
PS
The Proton Synchrotron, backbone of CERN’s accelerator complex.
Pseudo-rapidity
A quantity (denoted by η) frequently used in colliding beam experiments to express angles with respect to the axis of the colliding beams (typically for rays emanating from the center of the detector). It has the value 0 for rays perpendicular to the beam, and large positive or negative values for rays at small angles to the beam. The sign is chosen by convention: in CMS positive η refers to the end of the detector closer to the nearby Jura mountains.
Quadrupole
A magnet with four poles, used to focus particle beams rather as glass lenses focus light. There are 392 main quadrupoles in the LHC.
Quantum electrodynamics (QED)
The theory of the electromagnetic interaction.
Quantum chromodynamics (QCD)
The theory for the strong interaction, analogous to QED.
Quark
A class of elementary particle. Quarks are particles of matter that feel the strong force.
Quark–gluon plasma (QGP)
A new kind of plasma in which protons and neutrons are believed to break up into their constituent parts. QGP is believed to have existed just after the Big Bang.
Quench
A quench occurs in a superconducting magnet when the superconductor warms up and ceases to superconduct.
R-parity
To maintain the conservation of baryon and lepton number, a discrete multiplicative symmetry of R-parity is often imposed in SUSY models. This term is defined by R = (-1)2S+3B+L, with spin S, baryon number B and lepton number L.
Ring Imaging CHerenkov (RICH) counter
A kind of particle detector that uses the light emitted by fast-moving particles as a means of identifying them.
Scintillation
The flash of light emitted by an electron in an excited atom falling back to its ground state.
Sextupole
A magnet with six poles, used to apply corrections to particle beams. At the LHC, eight- and ten-pole magnets will also be used for this purpose.
Spectrometer
In particle physics, a detector system containing a magnetic field to measure momenta of particles.
Spin
Intrinsic angular momentum - a fundamental property of particles as described by quantum mechanics.
SPS
The Super Proton Synchrotron. An accelerator that provides beams for experiments at CERN, as well as preparing beams for the LHC.
Standard Model
A collection of theories that embodies all of our current understanding about the behaviour of fundamental particles.
Strong force
The strong force holds quarks together within protons, neutrons and other particles. It also prevents the protons in the nucleus from flying apart under the influence of the repulsive electrical force between them (because they all have positive charge). Unlike the more familiar effects of gravity and electromagnetism where the forces become weaker with distance, the strong force becomes stronger with distance.
Superconductivity
A property of some materials, usually at very low temperatures, that allows them to carry electricity without resistance. If you start a current flowing in a superconductor, it will keep flowing forever—as long as you keep it cold enough.
Superfluidity
A phase of matter characterized by the complete absence of resistance to flow.
Supersymmetry
A hypothesis that predicts the existence of heavy ‘superpartners’ to all known particles. It will be tested at the LHC.
Synchrotron
A particle accererator in which a magnetic field bends the orbits of the particles, which increases their energy. The particles travel in a circular path.
Technical Design Report (TDR)
The blueprint for an LHC sub-detector system.
Technology transfer
The promotion and dissemination to third parties of technologies developed, for example at CERN, for socio-economic and cultural benefits.
Transfer line
Carries a beam of particles, e.g., protons, from one accelerator to another using magnets to guide the beam.
Transverse momentum (and energy)
The amount of a particle's momentum which is perpendicular to the beam direction. Transverse momentum carried by unobserved particles such as neutrinos and dark matter particles is called "missing" transverse momentum; it can be inferred from the observed transverse momenta. For technical reasons a closely related quantity called transverse energy is often calculated instead of transverse momentum.
Trigger
An electronic system for spotting potentially interesting collisions in a particle detector and triggering the detector’s read-out system to record the data resulting from the collision.
Vacuum
A volume of space that is substantively empty of matter, so that gaseous pressure is much less than standard atmospheric pressure.
Weak force
The weak force acts on all matter particles and leads to, among other phenomena, the decay of neutrons (which underlies many natural occurrences of radioactivity) and allows the conversion of a proton into a neutron (responsible for hydrogen burning in the centre of stars). It can be either an attractive or a repulsive force.
Higgs Boson
Higgs Boson lucas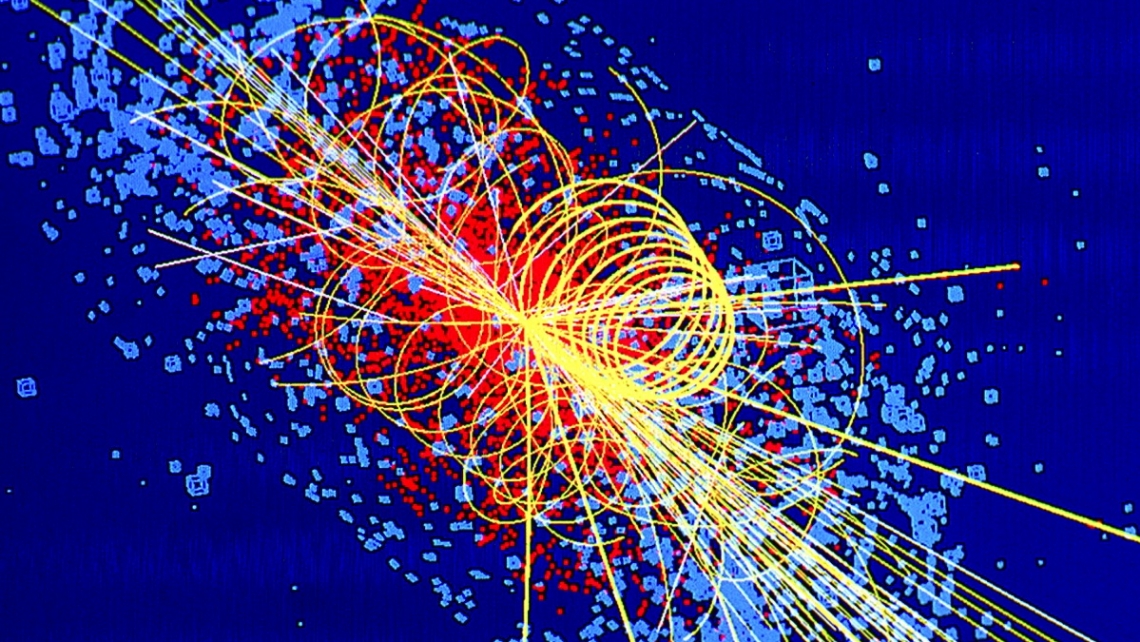
The Higgs boson is the fundamental particle predicted by the Brout-Englert-Higgs mechanism. This theory explains how fundamental particles acquire their mass. The search for the Higgs boson is one of the most fascinating scientific adventures. It started about 50 years ago and was considered impossible for decades, which is one of the main reasons the LHC was built.
The need for the Higgs
Our Universe seems to be described by four fundamental forces: gravity, electromagnetism, the weak force (which regulates nuclear phenomena like fusion within stars), and the strong force (which keeps atomic nuclei together). From around the turn of the last century, physicists have tried to unify these forces under a single, overarching theory. A major breakthrough occurred five decades ago when physicists realized that there are very close ties between the weak and electromagnetic forces. These two forces can be described by a single theory: electricity, magnetism, light, and some types of radioactivity are all manifestations of a single underlying force called the electroweak force.
The theory of electroweak interactions and Quantum Chromodynamics (the theory of the strong force) form the basis of the Standard Model. The Standard Model successfully describes all of the elementary particles we know and how they interact with one another. But our understanding of Nature is incomplete. In particular, the Standard Model as originally conceived could not explain one basic observation: why most elementary particles have mass. The symmetry responsible for electroweak unification requires the force-carrying particles involved to have no mass. The photon, carrier of the electromagnetic force, is found to follow that precept; however, the W and Z bosons, carriers of the weak force, have a mass. The fact that the W and Z boson are massive breaks the fundamental electroweak symmetry. It also leads, without corrections, to nonsensical predictions – for example, interactions with probabilities greater than 100%.
Needing a way out of this problem, several physicists[1] proposed a mechanism to account for the broken symmetry. Once incorporated into the equations, this electroweak-symmetry breaking mechanism would allow particles to have mass. The mechanism also explains why the weak interactions appear weak at low energies; the force carriers are massive, and therefore the force is correspondingly short-ranged. The Brout-Englert-Higgs mechanism requires the existence of a new particle, which we now call the Higgs boson.
According to our current understanding, all particles were massless just after the Big Bang. As the Universe cooled and the temperature fell below a critical value, an invisible field called the ‘Higgs field’ was formed; this field prevails throughout the cosmos. Particles such as the W and Z bosons acquire mass through their interaction with this field – the more intensely they interact, the heavier they become. The existence of the Brout-Englert-Higgs field explains the difference between the massless photon and the massive W and Z boson as we observe in Nature today. Other force-carrying particles – the photon and the gluon – do not have an interaction with the Higgs field and remain massless. The Higgs boson is the quantum particle associated with the Higgs field, just as the photon is the quantum particle associated with the electromagnetic field. Since the field cannot be observed directly, the LHC experiments search for its manifestation, the particle. The discovery of the particle proves the existence of the field.
Where does all mass come from?
Interactions with the Higgs field are not just reserved for force-carrying particles. The theory was extended to the rest masses of all other fundamental particles – such as the electron, or the quarks inside protons and neutrons. Composite particles, however, such as the protons and neutrons themselves, gain mass mainly through the binding energy holding them together. Without mass, the universe would be a very different place. For example, if the electron had no mass, there would be no atoms. Hence there would be no complex matter as we know it, no molecules, no chemistry, no biology, and no people. |
The particle hunt
The Higgs boson is the only fundamental particle predicted by the Standard Model that had not yet been seen by experiments when the LHC started. As for the other particles, the Standard Model does not predict the exact mass of the Higgs boson. Moreover, the probability of producing a Higgs boson is very low, making the particle more difficult to pin down. We had to look for it by systematically searching over a very large range of possible mass values. Fortunately, depending on its mass, the Standard Model Higgs boson was expected to leave characteristic footprints. The CMS experiment was built as a general purpose detector also for this purpose: to be sensitive to any possible Higgs mass. So we knew what to look for and starting from the measured particles in the detector, estimate its mass. If it turned out that we could not find it, this would have left the field wide open for physicists to develop a completely new theory to explain the origin of particle mass. The Higgs boson was at the top of physicists’ most-wanted list for more than four decades. However, in its most basic form, incorporating the Higgs field into the Standard Model is not completely satisfying. It does the job of explaining how the symmetry between electromagnetic and weak force-carriers is broken and it accounts for how force-carriers acquire their mass; but it does not predict or explain the degree of interaction with the field and hence the relative masses of these particles. Moreover, it does not explain why symmetry is broken in this way, nor does it predict the pattern of masses of quarks and leptons.
Other bosons – Looking beyond the Standard Model
We might find that the Higgs boson is different from the simplest version the Standard Model predicts. Many theories that describe physics beyond the Standard Model, such as Supersymmetry and compositeness models, suggest the existence of a zoo of new particles, including different kinds of Higgs bosons. CMS is a general-purpose detector. This means that not only is it designed with particular hypotheses in mind, but also with the aim to study whatever happens when particles collide at high energies, even if the results are like nothing we expect. If unexpected phenomena do occur, we are looking for them. It seems we are looking at just the visible tip of an iceberg – hidden below the Standard Model must be a deeper, more fundamental theory that gives reason to what we see on the surface.
References
[1] F. Englert and R. Brout, “Broken symmetry and the mass of gauge vector mesons”, Phys.Rev. Lett. 13 (1964) 321–323, doi:10.1103/PhysRevLett.13.321. P.W. Higgs, “Broken symmetries and the masses of gauge bosons”, Phys. Rev. Lett. 13 (1964) 508–509, doi:10.1103/PhysRevLett.13.508. G. Guralnik, C. Hagen, and T. W. B. Kibble, “Global conservation laws and massless particles”, Phys. Rev. Lett. 13 (1964) 585–587, doi:10.1103/PhysRevLett.13.585. It is also of note that Landau and Ginzburg had proposed a field giving the photon a mass in a superconductor, the maths of which is identical to the “Higgs mechanism” and predates it by several years. Credit: this text is based on a CERN "backgrounder" article and a CERN Bulletin article.
CMS Higgs Results
CMS Higgs Results lucasCMS Higgs Physics Results
Since the observation of the Higgs boson and establishing the couplings between the Higgs boson and photons, Z-bosons, and W-bosons, CMS physicists have made huge strides in the quest to better understand the Higgs boson.
With the data collected in 2016, we were able to observe the decay of the Higgs boson to tau leptons - strengthening previous results from combining CMS and ATLAS measurements that show the Higgs boson coupling to fermions (https://cds.cern.ch/record/2276465?ln=en )
Using the same data set, the production of the Higgs boson via top-antitop quark associated production was also confirmed, showing that the Higgs boson interacts with quarks as well as with leptons ( https://cms.cern/news/observation-tth-production )
In 2018, we observed the decay of the Higgs boson to pairs of b-quarks, completing the observation of the Higgs boson's interactions with all three of the heaviest fermions (https://cms.cern/news/higgs-observed-decaying-b-quarks-submitted )
Having confirmed the couplings between the Higgs boson and the three heaviest fermions, CMS also showed evidence for the interaction between the Higgs boson and the muon, the lighter cousin of the tau lepton. (https://cms.cern/news/cms-sees-evidence-higgs-boson-decaying-muons)
By combining measurements in the diphoton and four-lepton decay channels, we were also able to measure the mass of the Higgs boson with unprecedented precision of 0.1%! ( https://cms.cern/news/cms-precisely-measures-mass-higgs-boson - note that the article is based on a preliminary result; the published mass value is 125.38 ± 0.14 GeV)
Recently we have also measured the width of the Higgs boson by studying its decay into two Z-bosons: https://cms.cern/news/life-higgs-boson
ATLAS and CMS combine summer '11 search limits on the Standard Model Higgs
ATLAS and CMS combine summer '11 search limits on the Standard Model Higgs achintya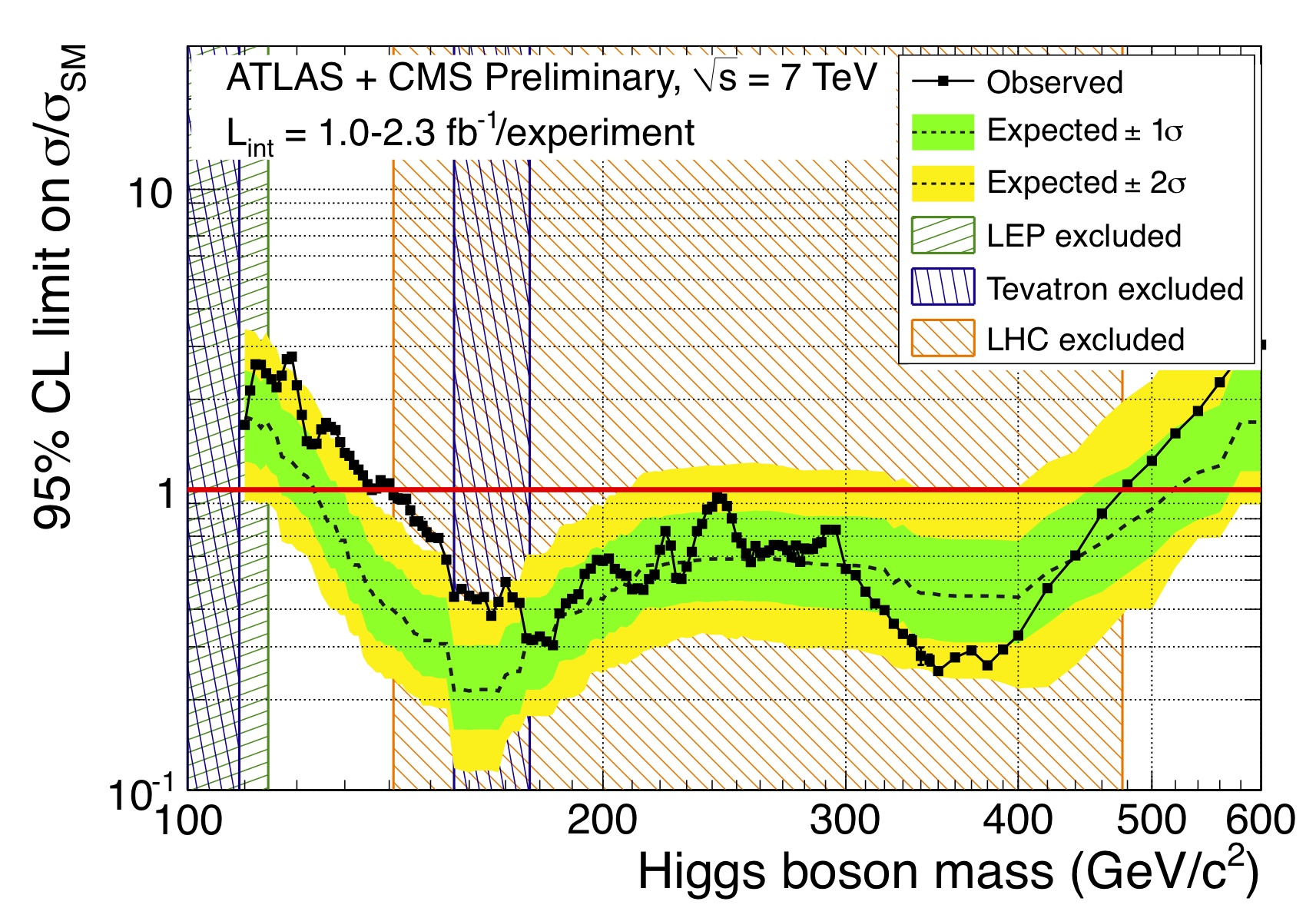
Perhaps the most anticipated result of the LHC involves the search for the Higgs boson, the only particle predicted by the Standard Model (SM) that has not yet been seen by experiments. The Higgs boson helps explain how elementary particles acquire mass. If the SM Higgs boson exists it will be produced at the LHC and swiftly decay into various known and well-studied particles, with the dominant decay products depending on the actual Higgs mass. ATLAS and CMS search for the SM Higgs boson using a range of decay products: two photons; two tau leptons; two b quarks; two W bosons; and two Z bosons. Analysing all these channels ensures that the search is sensitive to observing the Higgs irrespective of its mass. First results from ATLAS and CMS on searches for the SM Higgs boson with about 1 inverse femtobarn[1] (fb−1) of data were reported already in July at the EPS2011 meeting in Grenoble, and updates were shown one month later at the Lepton Photon 2011 conference in Mumbai with data samples of up to 2.3 fb−1. By combining the individual results of the two experiments an increased sensitivity in the search can be obtained. Hence, as a next logical step, the experiments have now combined the search data released this summer. A team of physicists from ATLAS and CMS was put in place early this year to prepare for such a combination. Correlations between the individual results have been carefully taken into account. Various statistical combination techniques have been explored to perform the combination, and a huge number of cross checks were made in the last few months. Finally, the combined search result is now ready. The results of the combination are shown in the figure, which depicts in a very clear way the enormous impact of the 2011 LHC data on the search for the SM Higgs boson. The CMS and ATLAS combined search excludes at >95% confidence level[2] the presence of the Standard Model Higgs in the mass range[3] 141-476 GeV. Indeed, the region from 146 to 443 GeV is excluded at 99% confidence level with the exception of three small regions between 220 and 320 GeV. Nonetheless, exploration continues of the region at low mass that is most favoured by indirect measurements, as the experiments collect and analyse more data to increase their sensitivity. Clearly this region will be the main focus of the searches with the complete 2011 data sample, which amounts to more than 5 fb−1 recorded by each of the experiments, and in 2012. At the same time, the search in the full range will continue to look for production of non-Standard Model Higgs bosons, with a possible lower production rate in the channels studied so far, and by exploring new decay channels. The next few months are definitely going to be very exciting. More information can be found in CMS-PAS-HIG-11-023, ATLAS-CONF-2011-157.
Video
Follow the combination process from the CMS perspective (includes exclusive footage from internal CMS meetings):
Additional information
- All CMS Higgs plots and results
- All CMS Papers
- All CMS Physics Analysis Summaries
- All CMS results
- Images of real collisions in CMS
- Animations of real collisions in CMS
About CMS
CMS is one of two general-purpose experiments at the LHC that have been built to search for new physics. It is designed to detect a wide range of particles and phenomena produced in the LHC's high-energy proton-proton and heavy-ion collisions and will help to answer questions such as: "What is the Universe really made of and what forces act within it?" and "What gives everything substance?" It will also measure the properties of well-known particles with unprecedented precision and be on the lookout for completely new, unpredicted phenomena. Such research not only increases our understanding of the way the Universe works, but may eventually spark new technologies that change the world in which we live as has often been true in the past. The conceptual design of the CMS experiment dates back to 1992. The construction of the gigantic detector (15 m diameter by nearly 29 m long with a weight of 14000 tonnes) took 16 years of effort from one of the largest international scientific collaborations ever assembled: more than 3100 scientists and engineers from 169 institutions and research laboratories distributed in 39 countries all over the world. For further information, contact: cms.outreach@cern.ch.
- [1] http://news.stanford.edu/news/2004/july21/femtobarn-721.html
http://www.quantumdiaries.org/2011/03/02/why-don%E2%80%99t-we-just-say-collision-rate/ - [2] Confidence level is a statistical measure of the number of times out of 100 that test results can be expected to be within a specified range. For example, a confidence level of 95% means that the result of an action will probably meet expectations 95% of the time. (Source: NADbank)
- [3] By mass-energy equivalence, the electron volt is also a unit of mass. It is common in particle physics, where mass and energy are often interchanged, to use eV/c2, where c is the speed of light in a vacuum (from E = mc2). Even more common is to use a system of natural units with c set to 1 (hence, E = m), and simply use eV as a unit of mass. (Source: Wikipedia)
CMS search for the Standard Model Higgs Boson in LHC data from 2010 and 2011
CMS search for the Standard Model Higgs Boson in LHC data from 2010 and 2011 lucas![Figure 1: A typical candidate event including two high-energy photons whose energy (depicted by dashed yellow lines and red towers) is measured in the CMS electromagnetic calorimeter. The yellow lines are the measured tracks of other particles produced in the collision. <a href="https://cdsweb.cern.ch/record/1406073">[ See more event display images. ]</a>](/sites/default/files/field/image/gg-run177878-evt188723900-3d.jpg)
CERN, 13th December 2011
The statement below is also available as PDF files in: Chinese (traditional) | Chinese (simplified) | Croatian | Dutch | English | Finnish | French | German | Greek | Hungarian | Italian | Persian | Polish | Spanish | Portuguese | Russian | Serbian | Turkish
The Higgs boson is the only particle predicted by the Standard Model (SM) of particle physics that has not yet been experimentally observed. Its observation would be a major step forward in our understanding of how particles acquire mass. Conversely, not finding the SM Higgs boson at the LHC would be very significant and would lead to a greater focus on alternative theories that extend beyond the Standard Model, with associated Higgs-like particles. Today the CMS Collaboration presented their latest results in the search for the Standard Model Higgs boson, using the entire data sample of proton-proton collisions collected up to the end of 2011. These data amount to 4.7 fb-1 of integrated luminosity[1], meaning that CMS can study Higgs production in almost the entire mass range above the limit from CERN’s Large Electron Positron (LEP) collider of 114 GeV/c2 (or 114 GeV in natural units [2]) and up to 600 GeV. Our results were achieved by combining searches in a number of predicted Higgs “decays channels” including: pairs of W or Z bosons, which decay to four leptons; pairs of heavy quarks; pairs of tau leptons; and pairs of photons (Figure 1). Our preliminary results, for several statistical confidence levels [3], exclude the existence of the SM Higgs boson in a wide range of possible Higgs boson masses:
- 127 – 600 GeV at 95% confidence level, as shown in Figure 2a; and
- 128 – 525 GeV at 99% confidence level.
A mass is said to be “excluded at 95% confidence level” if the Standard Model Higgs boson with that mass would yield more evidence than that observed in our data at least 95% of the time in a set of repeated experiments. We do not exclude a SM Higgs boson with a mass between 115 GeV and 127 GeV at 95% confidence level. Compared to the SM prediction there is an excess of events in this mass region (see Figure 2b), that appears, quite consistently, in five independent channels. With the amount of data collected so far, it is inherently difficult to distinguish between the two hypotheses of existence vs non-existence of a Higgs signal in this low mass region. The observed excess of events could be a statistical fluctuation of the known background processes, either with or without the existence of the SM Higgs boson in this mass range. The larger data samples to be collected in 2012 will reduce the statistical uncertainties, enabling us to make a clear statement on the possible existence, or not, of the SM Higgs boson in this mass region. The excess is most compatible with an SM Higgs hypothesis in the vicinity of 124 GeV and below, but with a statistical significance of less than 2 standard deviations (2σ) from the known backgrounds, once the so-called Look-Elsewhere Effect [4] has been taken into account. This is well below the significance level that traditionally has been associated with excesses that stand the test of time. If we explore the hypothesis that our observed excess could be the first hint of the presence of the SM Higgs boson, we find that the production rate (“cross section” relative to the SM, σ/σSM) for each decay channel is consistent with expectations, albeit with large uncertainties. However, the low statistical significance means that this excess can reasonably be interpreted as fluctuations of the background. More data, to be collected in 2012, will help ascertain the origin of the excess. UPDATE: CMS publishes Higgs boson search results using 2010–2011 data
- This statement is available online at:
http://cern.ch/cms/news/cms-search-standard-model-higgs-boson-lhc-data-2010-and-2011 - For more details see the CMS public website: http://cern.ch/cms
Event images and animations of real CMS data
- Collision event display images from the Higgs analysis of 2010 and 2011 data
- Collision event animation (4 electrons) from the Higgs analysis of 2010 and 2011 data
- Collision event animation (2 photon) from the Higgs analysis of 2010 and 2011 data
- Collision event animation (4 muons) from the Higgs analysis of 2010 and 2011 data
Short movies about the Higgs
- Animation of the Higgs mechanism [ with subtitles] See also [ without subtitles]
- What is the Higgs? by Don Lincoln
- Higgs Boson: How do we search for it? by Don Lincoln
Seminar at CERN, 13th December 2011
- CMS seminar slides (Prof. G. Tonelli)
- CERN Press Release, 13th December 2011 [ Also available in French ]
- ATLAS Experiment Higgs results
More about the Higgs at CMS
- CMS Physics Analysis summary papers about the Higgs
- Simple explanations of Higgs search terminology
- Search for the Standard Model Higgs Boson in CMS
- All CMS Higgs plots and results
About CMS Physics
- All CMS Papers
- All CMS Physics Analysis Summaries
- All CMS results
- Images of real collisions in CMS
- Animations of real collisions in CMS
About CMS
More information may be found on the CMS web site: http://cern.ch/cms. CMS is one of two general-purpose experiments at the LHC that have been built to search for new physics. It is designed to detect a wide range of particles and phenomena produced in the LHC's high-energy proton-proton and heavy-ion collisions and will help to answer questions such as: "What is the Universe really made of and what forces act within it?" and "What gives everything substance?" It will also measure the properties of well-known particles with unprecedented precision and be on the lookout for completely new, unpredicted phenomena. Such research not only increases our understanding of the way the Universe works, but may eventually spark new technologies that change the world in which we live as has often been true in the past. The conceptual design of the CMS experiment dates back to 1992. The construction of the gigantic detector (15 m diameter by nearly 29 m long with a weight of 14000 tonnes) took 16 years of effort from one of the largest international scientific collaborations ever assembled: more than 3100 scientists and engineers from 169 institutions and research laboratories distributed in 39 countries all over the world. For further information, contact: cms.outreach@cern.ch.
Footnotes
- [1] http://news.stanford.edu/news/2004/july21/femtobarn-721.html
- [2] The electron volt is a unit of energy. In particle physics, where mass and energy are often interchanged, it is common to use eV/c2 as a unit of mass (from E = mc2, where c is the speed of light in vacuum). Even more common is to use a system of natural units with c set to 1 (hence, E = m), and simply use eV as a unit of mass.
- [3] Confidence level is a statistical measure of the percentage of test results that can be expected to be within a specified range. For example, a confidence level of 95% means that the result of an action will probably meet expectations 95% of the time.
- [4] http://cmsexperiment.web.cern.ch/news/should-you-get-excited-your-data-…
New CMS Higgs Search Results for the Lepton Photon 2011 Conference
New CMS Higgs Search Results for the Lepton Photon 2011 Conference lucas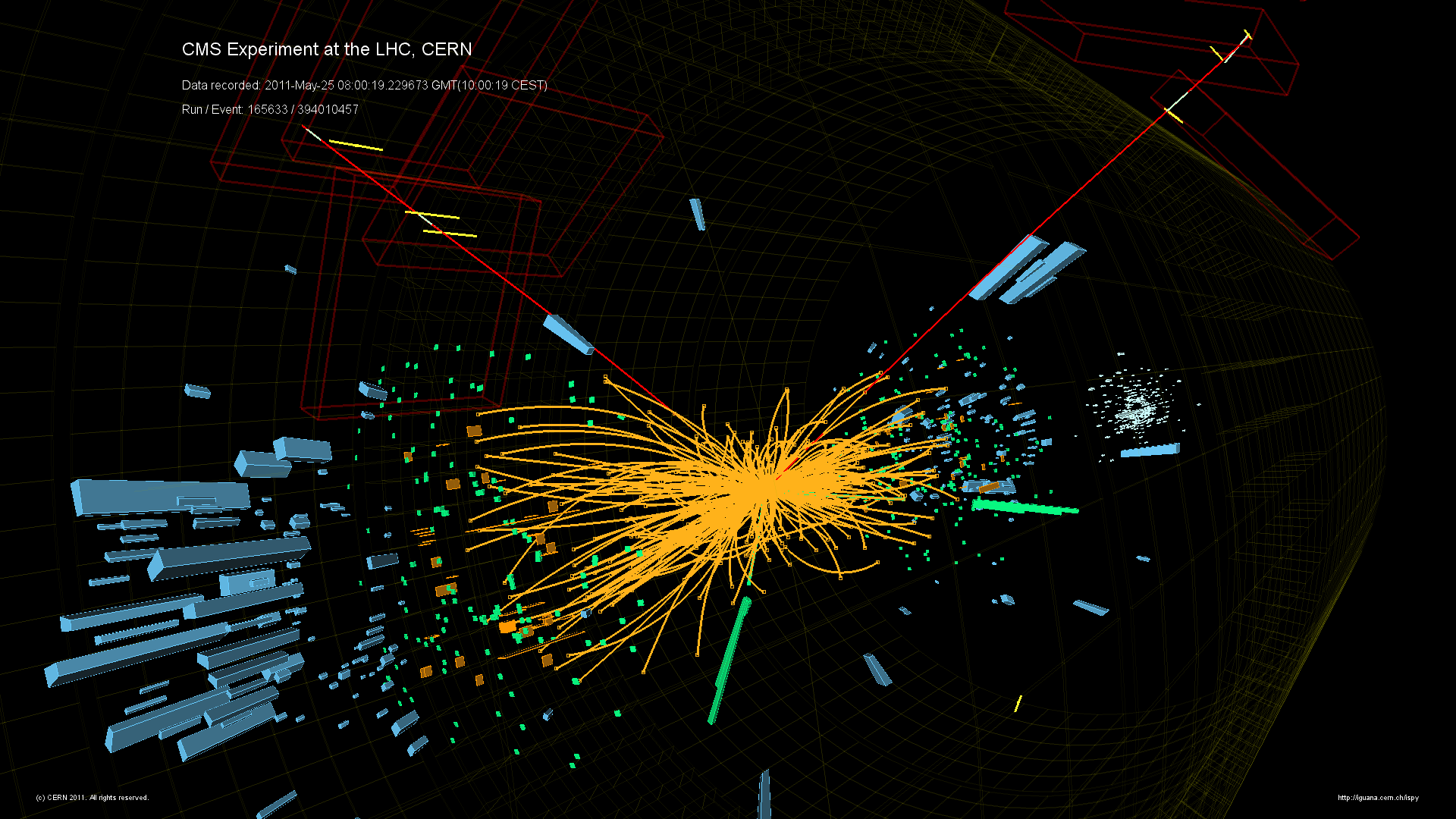
The Higgs boson is the only particle predicted by the Standard Model that has not yet been seen by experiments. It helps explain how elementary particles acquire mass. If the Higgs boson exists it will be produced in proton-proton collisions at the LHC. It then swiftly decays into various known and well-studied particles (depending on the actual Higgs mass). The CMS search for the Higgs boson is being carried out using a range of decay products: two photons; two tau leptons; two b quarks; two W bosons; and two Z bosons. Analysing all these channels ensures that the search is sensitive to observing the Higgs irrespective of its own mass. An example of an event passing the selection criteria is shown in Figure 1, in which two Z bosons are identified through their decays to a pair of electrons and a pair of muons respectively. These results are based on data recorded during LHC running at an energy of 7 TeV in 2010 and 2011. The analysis uses 1.1 – 1.7 inverse femtobarns[1] (integrated luminosity) of data, depending on the channel — an inverse femtobarn corresponds to about 100 trillion proton-proton collisions. CMS observes no convincing excess of events in the explored mass[2] range of 110-600 GeV (for full details see [HIG-11-022]). The analysis excludes, with a confidence level (C.L.) of 95%[3], the existence of a Standard Model Higgs boson in three Higgs mass ranges: 145-216 GeV, 226-288 GeV and 310-400 GeV (Figure 2). For the quantity of data we have collected, on average we would expect to exclude the range 130-440 GeV in the absence of a signal. We believe that the differences between the expected and observed exclusion mass ranges are consistent with statistical fluctuations. At 90% C.L., we exclude the SM Higgs boson in the mass range from 144-440 GeV. All exclusion regions were obtained using the modified frequentist construction confidence levels. It should be noted that a modest excess of events is observed for Higgs boson masses below 145 GeV. With the data we will collect in the coming months we will be able to distinguish between the possible interpretations: the production of a SM Higgs boson or a statistical fluctuation of the backgrounds. During the ongoing LHC proton-proton data-taking period, expected to terminate at the end of 2012, CMS will record substantially more data that should be sensitive to observing a SM Higgs boson, if it exists, over the full range of possible masses.
More information
- All CMS Papers (see also: timeline graphical index of all papers)
- All CMS Physics Analysis Summaries
- All CMS results
About CMS
More information, including images and animations of CMS collision events, may be found on the CMS web site: http://cms.cern.ch. CMS is one of two general-purpose experiments at the LHC that have been built to search for new physics. It is designed to detect a wide range of particles and phenomena produced in the LHC's high-energy proton-proton and heavy-ion collisions and will help to answer questions such as: "What is the Universe really made of and what forces act within it?" and "What gives everything substance?" It will also measure the properties of well-known particles with unprecedented precision and be on the lookout for completely new, unpredicted phenomena. Such research not only increases our understanding of the way the Universe works, but may eventually spark new technologies that change the world in which we live as has often been true in the past. The conceptual design of the CMS experiment dates back to 1992. The construction of the gigantic detector (15 m diameter by nearly 29 m long with a weight of 14000 tonnes) took 16 years of effort from one of the largest international scientific collaborations ever assembled: more than 3100 scientists and engineers from 169 institutions and research laboratories distributed in 39 countries all over the world. For further information, contact: cms.outreach@cern.ch.
- [1] http://news.stanford.edu/news/2004/july21/femtobarn-721.html
http://www.quantumdiaries.org/2011/03/02/why-don%E2%80%99t-we-just-say-collision-rate/ - [2] By mass-energy equivalence, the electron volt is also a unit of mass. It is common in particle physics, where mass and energy are often interchanged, to use eV/c2, where c is the speed of light in a vacuum (from E = mc2). Even more common is to use a system of natural units with c set to 1 (hence, E = m), and simply use eV as a unit of mass. (Source: Wikipedia)
- [3] Confidence level is a statistical measure of the number of times out of 100 that test results can be expected to be within a specified range. For example, a confidence level of 95% means that the result of an action will probably meet expectations 95% of the time. (Source: NADbank)
New CMS Results for the EPS 2011 Conference
New CMS Results for the EPS 2011 Conference lucas![Fig 1. Event display of a ZZ event. One Z decays to two electrons (red towers) [...]](/sites/default/files/field/image/Fig1-CMS-2e-2mu-evt-394010457-3D.jpg)
The CMS collaboration is presenting its latest results this week at the 2011 Europhysics Conference on High-Energy Physics, held in Grenoble, France. These results are based on about 1 inverse femtobarn[1] of data (100 trillion proton-proton collisions) from LHC running at an energy of 7 TeV, which were collected in 2010 and 2011. They include a wide range of searches for new physics and precise measurements of Standard Model processes.
Higgs Search
The Higgs boson is the only particle predicted by the Standard Model that has not yet been seen by experiments. It helps explain how elementary particles acquire mass. If the Higgs boson exists it will be produced in proton-proton collisions at the LHC and then swiftly decay into several known and well-studied particles. The CMS search for the Higgs boson is being carried out using a range of decay products: two photons; two tau leptons; two W bosons; and two Z bosons. The W is observed through its decay to an electron plus a neutrino, or a muon plus a neutrino. The Z is observed through its decay to a pair of electrons, or a pair of muons, (see Figure 1) or a pair of jets of hadronic particles. Analysing all these channels ensures that the search is sensitive to observing the Higgs irrespective of its own mass. CMS observes no convincing excesses of events in the explored mass[2] range of 120-600 GeV (for full details see [HIG-11-011]). The analysis excludes, with a confidence level of 95%[3], the existence of a Higgs boson in two broad Higgs mass ranges, 149-206 GeV and 300-440 GeV, as well as several narrower intervals in between (Figure 2). At a lower confidence level of 90%, the existence of a Higgs boson is excluded for the range 145-480 GeV. Re-interpreting the results in the context of the Standard Model with a fourth generation of fermions in addition to the known three generations (SM4), allows us to exclude the SM4 Higgs boson with a mass in the range 120-600 GeV with a confidence level of 95%. It should be noted that a modest excess of events is observed for Higgs boson masses below 145 GeV. With the data we will collect in the next few months we will be able to distinguish between the possible interpretations: the production of a Higgs boson or a statistical fluctuation of the backgrounds. During the ongoing LHC proton-proton run CMS will record substantially more data that should be sensitive to observing a Higgs boson, if it exists, over the full range of possible masses.
Search for rare decays of Bs→μμ
Bs mesons, comprising a 'bottom' and a 'strange' quark, are produced copiously at the LHC. The fraction that subsequently decays (known as the "branching fraction") to a pair of easily-detected muons is highly suppressed in the Standard Model — only about three such decays are expected per billion Bs particles produced. Several extensions of the SM, for instance Supersymmetric models, predict significant enhancements in the number of decays to pairs of muons, thanks to new particles that would contribute to the decay through "virtual loops". Therefore, any enhancement of this Bs decay rate could indicate the existence of new physics. CMS has searched for the decays of Bs (and B0 particles, comprising a 'bottom' and a 'down' quark) to muon pairs using proton-proton collision data collected up to June 2011 (Figure 3). A challenging aspect of this search is reducing the large backgrounds from other B-hadron decays or particles misidentified as muons. The number of candidate decays observed in the available data sample is consistent with the Standard Model expectations for signal and background (see Figure 4). Given the absence of a significant excess, CMS has excluded (at 95% confidence level) branching fractions larger than 1.9x10-8 and 4.6x10-9 for the decay of Bs and B0 particles, respectively. This result constitutes one of the most stringent exclusion limits achieved until now. The data CMS will collect in the remainder of 2011 and in 2012 will be sensitive to smaller branching fractions, at the level of SM expectations, and may lead to the observation of an enhanced decay rate which could be indicative of a non-Standard-Model physics process.
Other Results
CMS has searched for many other possible signatures of exotic new physics. No significant signals for new physics have yet been seen and therefore new constraints, at 95% confidence level, have been placed on many extensions to the Standard Model, including the following:
- The Supersymmetry (SUSY) theory provides a symmetry between matter and forces, and predicts that for each known particle there is a 'supersymmetric' partner. SUSY quarks, or squarks, are ruled out by CMS up to 1.2 TeV for a large range of SUSY parameters in the models tested [SUS-11-003].
- High-mass W′ and Z′ particles with Standard Model couplings are ruled out up to masses of 2.27 TeV [EXO-11-024] and 1.94 TeV [EXO-11-019] respectively. Constraints are placed on other models producing high-mass di-lepton resonances. Analysis of top-anti-top final states rules out the "Kaluza Klein gluon" (in Randal Sundrum models) with mass below 1.5 TeV [EXO-11-006].
- A study of di-jet resonances excludes excited quarks in the mass range of 1.0 TeV to 2.5 TeV and similarly constrains models involving E6 di-quarks, string resonances, the W′, axigluons and colorons [EXO-11-015].
- A search for the pair production of a fourth-generation t′ quark and its antiparticle ruled them out up to a t′ mass of 450GeV [EXO-11-051].
- Microscopic black-hole production is ruled out for black-hole masses up to between 4 and 5 TeV, depending on the model in question [EXO-11-071].
CMS also has a rich programme that confronts the predictions of the Standard Model with precise experimental measurements at the high-energy frontier of the LHC. These include top-quark production that has been studied in detail, with recent measurements made of the cross-section for top quark-top antiquark pairs decaying fully hadronically [TOP-11-007], the cross-section measurement using events with a tau lepton in the final state [TOP-11-006] and the top charge asymmetry [TOP-11-014]. Many aspects of the Electroweak and Strong forces as well as b-quarks are being studied. These analyses have already resulted in more than thirty publications in refereed journals, with many more results in the pipeline. A good understanding of Standard Model processes is also important as they contribute to the backgrounds to new physics processes.
Outlook
The LHC machine is running extremely well and has already delivered more proton-collision data than was expected in the entire 2011 run. The high-precision CMS experiment is recording good quality data with high efficiency. By the end of 2012 we expect to increase the size of our data sample by a factor of about ten. We are just starting to explore a vast territory where new physics could manifest itself and the CMS experiment is ready for whatever Nature has in store for us.
More information
- All CMS Papers (see also: timeline graphical index of all papers)
- All CMS Physics Analysis Summaries
- All CMS results
About CMS
More information, including images and animations of CMS collision events, may be found on the CMS web site: http://cms.cern.ch. CMS is one of two general-purpose experiments at the LHC that have been built to search for new physics. It is designed to detect a wide range of particles and phenomena produced in the LHC's high-energy proton-proton and heavy-ion collisions and will help to answer questions such as: "What is the Universe really made of and what forces act within it?" and "What gives everything substance?" It will also measure the properties of well-known particles with unprecedented precision and be on the lookout for completely new, unpredicted phenomena. Such research not only increases our understanding of the way the Universe works, but may eventually spark new technologies that change the world in which we live as has often been true in the past. The conceptual design of the CMS experiment dates back to 1992. The construction of the gigantic detector (15 m diameter by nearly 29 m long with a weight of 14000 tonnes) took 16 years of effort from one of the largest international scientific collaborations ever assembled: more than 3100 scientists and engineers from 169 institutions and research laboratories distributed in 39 countries all over the world. For further information, contact: cms.outreach@cern.ch.
- [1] http://news.stanford.edu/news/2004/july21/femtobarn-721.html
http://www.quantumdiaries.org/2011/03/02/why-don%E2%80%99t-we-just-say-collision-rate/ - [2] By mass-energy equivalence, the electron volt is also a unit of mass. It is common in particle physics, where mass and energy are often interchanged, to use eV/c2, where c is the speed of light in a vacuum (from E = mc2). Even more common is to use a system of natural units with c set to 1 (hence, E = m), and simply use eV as a unit of mass. (Source: Wikipedia)
- [3] Confidence level is a statistical measure of the number of times out of 100 that test results can be expected to be within a specified range. For example, a confidence level of 95% means that the result of an action will probably meet expectations 95% of the time. (Source: NADbank)
Higgs Boson -- Terms and Definitions
Higgs Boson -- Terms and DefinitionsBackground
When scientists search for new physics, they compare what they observe to what theories predict they will observe. The background is the set of results scientists expect to see. If an experiment sees more instances of a certain type of event (see “Excess”) than they expect to see as part of the background, it might be evidence of new physics.
Confidence Level (CL)
Confidence level is a statistical measure of the percentage of test results that can be expected to be within a specified range. For example, a confidence level of 95 percent means that the result of an action will probably meet expectations 95 percent of the time. When physicists search for a signal of the Higgs boson, they select particle collisions with observed characteristics similar to those a Higgs production would feature. The result of this data selection will always include background events, i.e. ones which did not involve a Higgs boson decay, but are anyway individually undistinguishable from the signal. The existence of the Higgs boson would however increase the number of selected events, so one may compare the number of selected events with the predicted yield from known backgrounds alone, to make a statistical statement about the Higgs production rate. If the number of observed events is close to the background prediction, then one can rule out a sizable contribution from the Higgs boson decay signal, which would have created an excess of events. Given a signal rate and a background prediction, one can compute the probability that a total number of events smaller or equal to the ones observed is seen. Signal rates for which the probability would be smaller than 5% are then said to be "excluded at 95% confidence level". Note that the choice of 95% as a division line between what is allowed or excluded by the data is arbitrary, and has merely historical justification.
Decay channel
Most massive particles like the Higgs boson are unstable and decay into other particles over time. Just as a vending machine might return the same amount of change using different combinations of coins, a particle can decay into different combinations of particles. These sets of secondary particles are called decay channels. If the Standard Model Higgs boson exists, it could decay into several different channels, such as two photons or two W bosons or two Z bosons. Physicists have calculated how often a Standard Model Higgs boson would decay into these different channels depending on its mass. They search for excesses of events in those decay channels, which could indicate the presence of a Higgs.
Event
An event is a snapshot of a collision in the LHC. Because energy is equivalent to mass, highly energetic collisions can create particles more massive than those involved in the collisions (protons, in the case of the LHC). These massive particles quickly decay into lighter stable particles (see “Decay channel”). Physicists study the decay products of collisions to determine what more massive particles were created in the events.
Excess
When scientists observe more of a certain type of event than expected in a data plot, they call that an excess. Scientists measure the statistical significance (See “Sigma” and “Standard deviation”) of excesses to determine how certain they are that they result from new physics and not simply random fluctuations.
Exclusion
If searches for a particle reveal statistically that it is unlikely to exist with certain characteristics (e.g. a particular mass), those characteristics can be excluded. This narrows the search parameters within which the particle might be found. Establishing such exclusions is important in the search for undiscovered particles.
Look-elsewhere effect (LEE)
When physicists see more of a certain type of event than predicted, they must consider the look-elsewhere effect in determining the statistical significance of that excess of events. To calculate the look-elsewhere effect for a certain observation, scientists take into account the probability of seeing something similar in any particular spot over the mass range in question. The chances of a statistical fluctuation causing an excess of events at one point on a plot are lower than the chances of a statistical fluctuation leading to an excess of at any point in a range of points on a plot. Other things being equal, the smaller the range one considers, the smaller the look-elsewhere effect. [Read more.]
Standard Deviation / sigma
The standard deviation describes the spread of a set of measurements around the mean value. It can be used to quantify the level of disagreement of a set of data from a given hypothesis. Physicists express standard deviations in units called “sigma”. The higher the number of sigma, the more incompatible the data are with the hypothesis. Typically, the more unexpected a discovery is, the greater the number of sigma physicists will require to be convinced.
Standard Model
The Standard Model is a collection of theories that embodies all of our current understanding about the behaviour of fundamental particles.
Blind analysis
To avoid bias when analysing new data, physicists perform the analysis “blind” — that is, without seeing how the application of various selection criteria would affect the result before the analysis is complete. [Read more.]
How is CMS searching for the Higgs Boson?
How is CMS searching for the Higgs Boson? lucas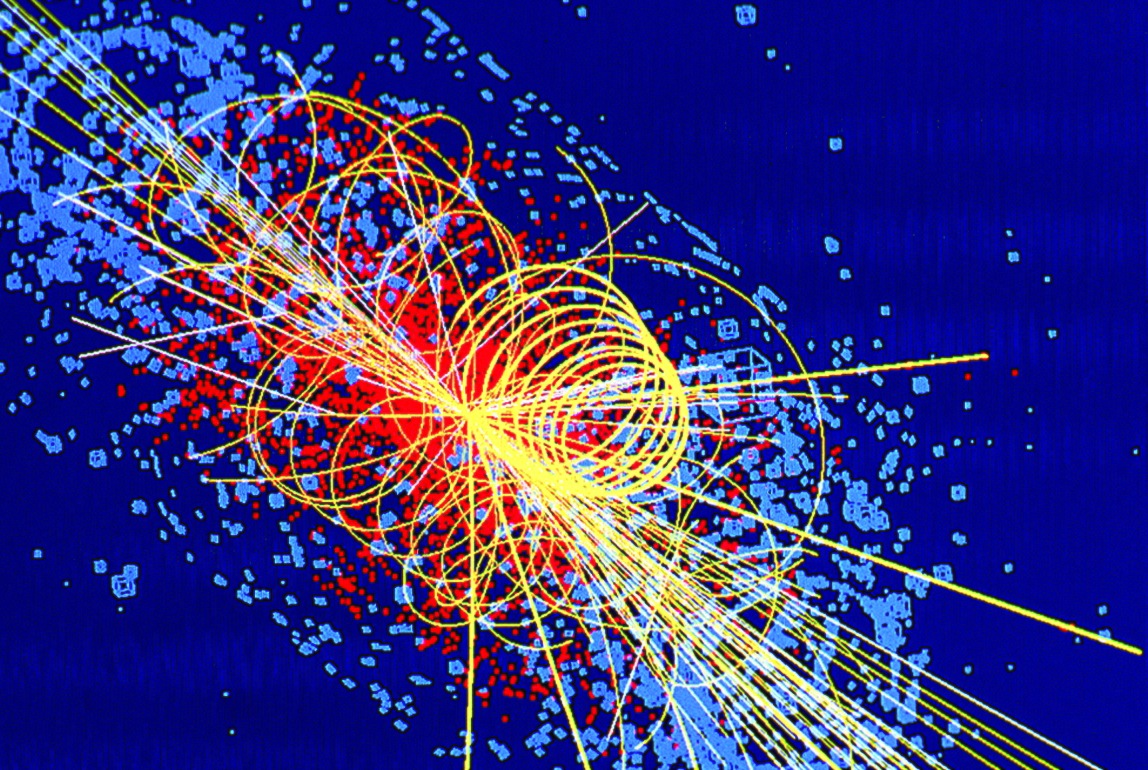
The discovery of the Higgs boson, one of the main goals of the CMS experiment, proved that the Higgs field exists. When a Higgs boson is formed in an LHC collision, it decays very quickly to other particles. Therefore, we have to study these decay products, the 'signature' the Higgs boson leaves behind, to learn about the Higgs boson. When the Higgs boson was first observed, it was seen in decays to pairs of Z-bosons, with subsequent decay into four leptons (particles such as electrons or muons), as well as in decays to pairs of photons.
The event shows characteristics expected from the decay of the SM Higgs boson to a pair of Z bosons, one of which subsequently decays to a pair of electrons (green lines and green towers) and the other Z decays to a pair of muons (red lines).
Photons are detected in the electromagnetic calorimeter. In the first image we see the detector head-on with the electromagnetic calorimeter (ECAL) in green. Green trajectories show these photons, while the blue trajectories show other particles emerging from the collision with the red marks showing hits in the hadron calorimeter. The ECAL is able to tell the mass of the particle to better than 1%.
Event recorded with the CMS detector in 2012 at a proton-proton centre of mass energy of 8 TeV. The event shows characteristics expected from the decay of the SM Higgs boson to a pair of photons (dashed yellow lines and green towers).
You can watch this video series to learn more about how the Higgs boson was discovered. https://www.youtube-nocookie.com/watch?v=so2nCu2Jkbc
Now that the Higgs boson has been discovered, at CMS, we're making further measurements of its properties. We're not just looking at the two discovery channels for this, but also at other major Higgs boson decay modes: into pairs of b-quarks, tau leptons, and W-bosons... If you'd like to know more, this video series sheds some more light on these different channels.
Blinding and unblinding analyses
Blinding and unblinding analyses achintya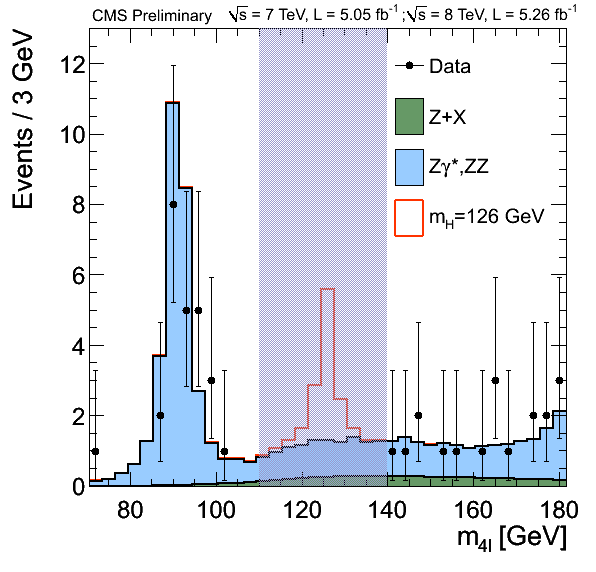
CMS performs searches for new particles by looking for signals amidst a background of known physics. If the data begin to indicate something more interesting than merely background - for instance, more decay events than expected in a certain region - it is important to make sure that the observation is statistically significant by collecting and analysing more data. However, we don't want to bias our analyses by optimising the analysis based on what was already seen. To avoid such bias while analysing new data, physicists draw "blinds” over the region where an excess of decay events is expected; this region is only "unblinded" when they are satisfied with their procedures. This ensures objectivity when it comes to looking for much-sought-after signs of new physics, and gives confidence in the ultimate result.
The procedure is similar to that used by medical researchers when testing a new treatment. Even though the Higgs boson was discovered a decade ago, we still employ this procedure for measuring the Higgs boson's properties - we still do not want to bias these measurements by optimising towards deviations from the standard model expectations! All of the available data, including those in the blinded region, are used in the analysis; you just don’t peek at this region to see how it changes as you tweak various selection criteria. In many of the on-going searches and measurements, data corresponding to a possible signal look very similar to data corresponding to background.
Therefore, once the analysis is blinded, physicists must optimise two things: 1) simulations, to help determine the criteria for selecting signals and 2) the methods for rejecting or quantifying background events using data from the region where there is no excess. To cross-check all the details, the various steps involved in the analysis procedures are usually carried out by at least two independent teams. If both teams see similar results in the background region, the analysis is signed off by the wider collaboration. It is only after the sign-off that the signal region is unblinded. The results of the unblinding are put through further scrutiny by the collaboration before being made public.
Should you get excited by your data? Let the Look-Elsewhere Effect decide
Should you get excited by your data? Let the Look-Elsewhere Effect decide dorigo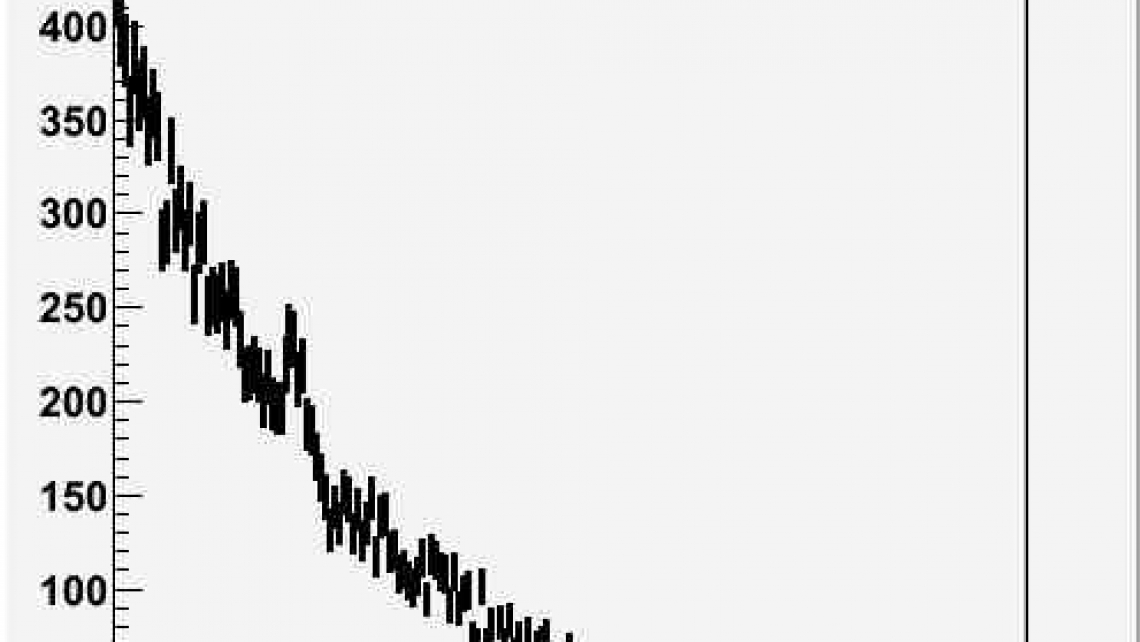
A dangerous beast is hiding in today’s searches for the rare signs of new physics — or even “old” physics, such as the Higgs boson — at the Large Hadron Collider. It is called “Look-Elsewhere Effect”, LEE for insiders. What is it, and why should you care? Imagine you look for a heavy particle decaying into a pair of hadronic jets: a commonplace test case in high-energy physics. You have your background model, which predicts the observable shape of the di-jet mass distribution, and you know what kind of a bump a new particle signal would produce in that shape. So you search for such a bump in the data, but — not knowing where it might appear — you search everywhere. You have worked all day, and the night is nearing; you prepare yourself a martini and spin your analysis program. To your amazement, the program finds a significant bump at some particular mass value: is it a real signal? To claim it is a new signal, the effect must reach or exceed the “five-sigma” significance level, five standard deviations away from the expectation: that's a rather silly but well-established rule. But if yours gives only 3.5 or four sigma, are you allowed to get excited and wake up your boss, or should you sit back and sip your martini, with an “I know better” grin on your face? I claim the latter is a better option. You have fallen prey to the LEE: you looked in many places for a possible signal, and found a significant effect somewhere; the likelihood of finding something in the entire region you probed is greater than it would be if you had stated beforehand where the signal would be, because of the “probability boost” of looking in many places. A good rule of thumb is the following: if your signal has a width W, and if you examined a spectrum spanning a mass range from M1 to M2, then the “boost factor” due to the LEE is (M2-M1)/W. This may easily amount to a factor of 10 or 100, depending on the details of your search. An effect occurring by chance once in ten thousand cases in a given place on your spectrum may actually be just an unexciting one-in-a-hundred fluctuation! In fact, the “five-sigma” rule I mentioned above was conceived with exactly this particular effect in mind. Five sigma is a really, really rare occurrence (three in ten million), so even including the LEE, it is still something to take quite seriously. Now-a-days, we publish three-sigma results that may or may not go away with more data, and our scientific integrity requires us to account for the LEE. This is actually less easy to do than by just multiplying a probability by (M2-M1)/W as in the example above: in complex searches such as that for the Higgs boson, which combine bump hunts in many channels, this is not a trivial task. A recent paper by E. Gross and O. Vitells (Eur. Phys. J. C70:525-530,2010) has clarified some of the technical issues. The searches for the Higgs boson by CMS and ATLAS are sizing up the LEE by studying the probability of the background-only hypothesis as a function of the Higgs mass: the more the observed p-value distribution varies up and down as the signal mass hypothesis change, the stronger is the Look-Elsewhere-Effect correction that is required. Stuff for experts, for sure. But outsiders have better be aware that a three-sigma effect should not be blindly dubbed “evidence” for something new in the data. As travellers to a foreign country whose tax habits are unknown, you better ask before you buy, “LEE included or not”? — Submitted by Tommaso Dorigo
Observation of a New Particle with a Mass of 125 GeV
Observation of a New Particle with a Mass of 125 GeV lucas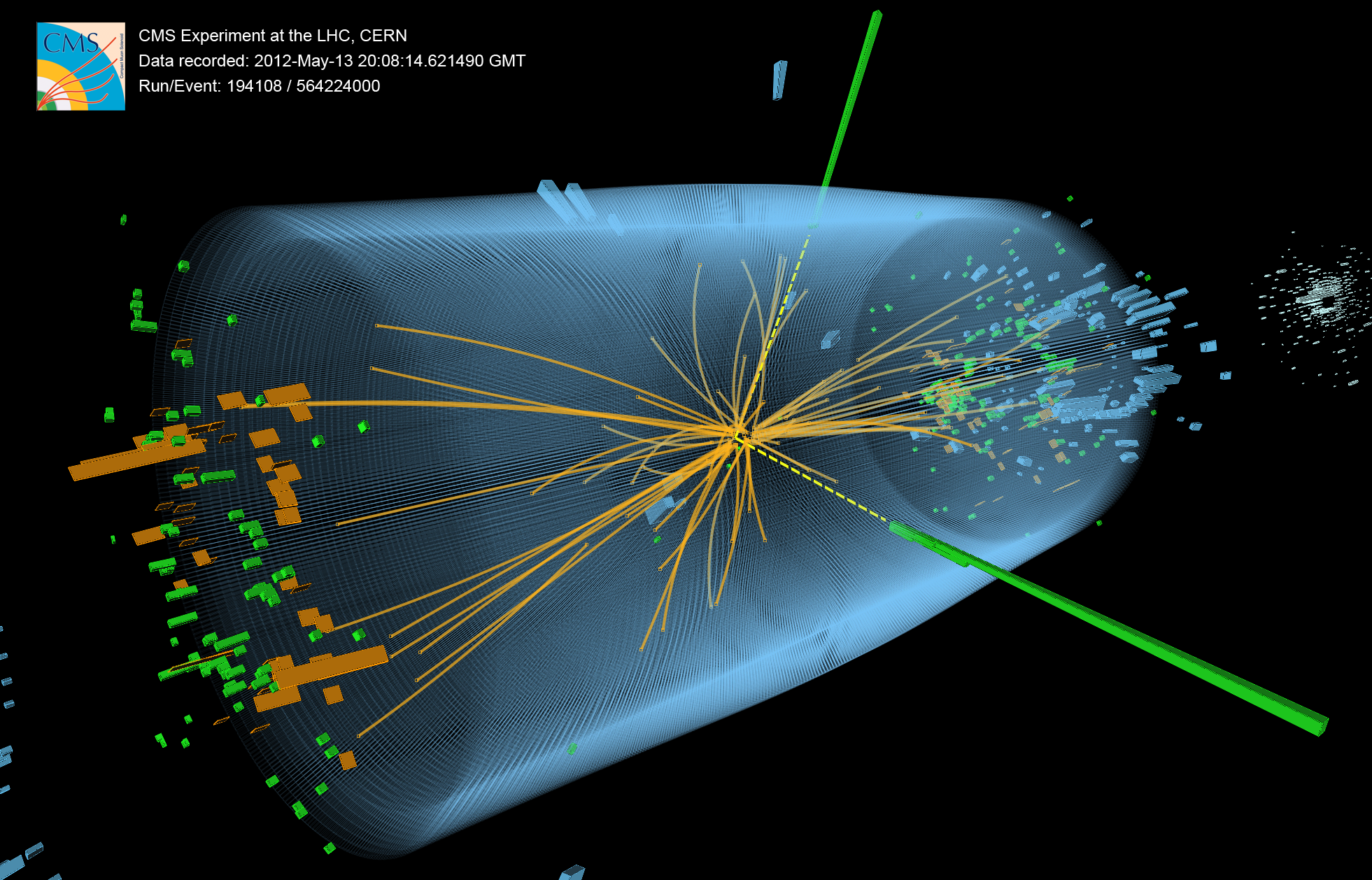
CMS Experiment, CERN
4 July 2012
The statement below is also available as PDF files in: English | French | Catalan | Chinese (traditional) | Chinese (simplified) | Croatian | Dutch | Finnish | Galician | German | Greek | Hindi | Hungarian | Italian | Korean | Persian | Polish | Portuguese | Romanian | Russian | Serbian | Spanish | Mexican Spanish | Turkish | Urdu
Summary
In a joint seminar today at CERN and the “ICHEP 2012” conference[1] in Melbourne, researchers of the Compact Muon Solenoid (CMS) experiment at the Large Hadron Collider (LHC) presented their preliminary results on the search for the standard model (SM) Higgs boson in their data recorded up to June 2012. CMS observes an excess of events at a mass of approximately 125 GeV[2] with a statistical significance of five standard deviations (5 sigma)[3] above background expectations. The probability of the background alone fluctuating up by this amount or more is about one in three million. The evidence is strongest in the two final states with the best mass resolution: first the two-photon final state and second the final state with two pairs of charged leptons (electrons or muons). We interpret this to be due to the production of a previously unobserved particle with a mass of around 125 GeV. The CMS data also rule out the existence of the SM Higgs boson in the ranges 110–122.5 GeV and 127–600 GeV with 95% confidence level[4] – lower masses were already excluded by CERN’s LEP collider at the same confidence level. Within the statistical and systematic uncertainties, results obtained in the various search channels are consistent with the expectations for the SM Higgs boson. However, more data are needed to establish whether this new particle has all the properties of the SM Higgs boson or whether some do not match, implying new physics beyond the standard model. The LHC continues to deliver new data at an impressive rate. By the end of 2012, CMS hopes to have more than triple its total current data sample. These data will enable CMS to elucidate further the nature of this newly observed particle. They will also allow CMS to extend the reach of their many other searches for new physics.
CMS Search Strategy
CMS analysed the full data sample of proton-proton collisions collected in all of 2011 and in 2012, up until June 18. These data amount to up to 5.1 fb−1 of integrated luminosity[5], at a centre-of-mass energy of 7 TeV in 2011 and up to 5.3 fb−1 at 8 TeV in 2012. The standard model predicts that the Higgs boson lasts for only a very short time before it breaks up, or “decays”, into other well-known particles. CMS studied five main Higgs boson decay channels. Three channels result in pairs of bosonic particles (γγ, ZZ or WW) and two channels result in pairs of fermionic particles (bb or ττ), where γ denotes a photon, Z and W denote the force carriers of the weak interaction, b denotes a bottom quark, and τ denotes a tau lepton. The γγ, ZZ and WW channels are equally sensitive in the search for a Higgs boson around 125 GeV and all are more sensitive than the bb and ττ channels. The γγ and ZZ channels are especially important as they both allow the mass of the new particle to be measured with precision. In the γγ channel the mass is determined from the energies and directions of two high-energy photons measured by the CMS crystal electromagnetic calorimeter (ECAL, Figure 1). In the ZZ channel the mass is determined from the decays of the two Zs to two pairs of electrons, or two pairs of muons, or a pair of electrons and a pair of muons (Figure 2). These are measured in the ECAL, inner tracking and muon detectors. The WW channel is more complex. Each W is identified through its decay to an electron and a neutrino or a muon and a neutrino. The neutrinos pass through the CMS detectors undetected, so the SM Higgs boson in the WW channel would manifest itself as a broad excess in the mass distribution, rather than a narrow peak. The bb channel has large backgrounds from standard model processes, so the analysis searches for events in which a Higgs boson is produced in association with a W or Z, which then decays to electron(s) or muon(s). The ττ channel is measured by observing τ decays to electrons, muons and hadrons.
CMS Search Results
The CMS data sample should be sensitive enough to completely exclude the mass range 110–600 GeV at 95% confidence level, if the SM Higgs does not exist. In fact, the CMS data do rule out the existence of the SM Higgs boson in two broad mass ranges of 110–122.5 GeV and 127–600 GeV with 95% confidence level. The range of 122.5–127 GeV cannot be excluded because we see an excess of events in three of the five channels analysed:
- γγ channel: the γγ mass distribution is shown in Figure 3. There is an excess of events above background with a significance of 4.1 sigma, at a mass near 125 GeV. The observation of the two-photon final state implies that the new particle is a boson, not a fermion, and that it cannot be a “spin 1” particle.
- ZZ channel: Figure 4 shows the mass distribution for the four leptons (two pairs of electrons, or two pairs of muons, or the pair of electrons and the pair of muons). Accounting also for the decay angle characteristics, it yields an excess of 3.2 sigma above background at a mass near 125 GeV.
- WW channel: a broad excess in the mass distribution of 1.5 sigma is observed.
- bb and ττ channels: no excess is observed.
The statistical significance of the signal, from a combined fit to all five channels (Figure 5), is 4.9 sigma above background. A combined fit to just the two most sensitive and high-resolution channels (γγ and ZZ) yields a statistical significance of 5.0 sigma. The probability of the background alone fluctuating up by this amount or more is about one in three million. The mass of the new particle is determined to be 125.3 ± 0.6 GeV, independent of any assumptions about the expected relative yields of the decay channels. The measured production rate (σDAT) of this new particle is consistent with the predicted rate (σSM) for the SM Higgs boson: σDAT/σSM = 0.80 ± 0.22. Great care has also been taken to understand numerous details of the detector performance, the event selection, background determinations and other possible sources of systematic and statistical uncertainties. The 2011 analysis[6] showed an excess of events at about 125 GeV. Therefore, to avoid a potential bias in the choice of selection criteria for the 2012 data that might artificially enhance this excess, the 2012 data analysis was performed “blind”[7], meaning that the region of interest was not examined until after all the analysis criteria had been fully scrutinized and approved. As a general cross-check, the analyses were performed by at least two independent teams. A number of other features reinforce confidence in the results:
- The excess is seen at around 125 GeV in both the 2011 data sample (7 TeV) and the 2012 data sample (8 TeV);
- The excess is seen at the same mass in both the high-resolution channels (γγ and ZZ);
- The excess seen in the WW is consistent with one that would arise from a particle at 125 GeV;
- The excess is seen in a range of final states involving photons, electrons, muons and hadrons.
The preliminary results presented today will be refined, with the aim of submitting them for publication towards the end of the summer.
Future Plans
The new particle observed at about 125 GeV is compatible, within the limited statistical accuracy, with being the SM Higgs boson. However, more data are required to measure its properties such as decay rates in the various channels (γγ, ZZ, WW, bb and ττ) and ultimately its spin and parity, and hence ascertain whether it is indeed the SM Higgs boson or the result of new physics beyond the standard model. The LHC continues to perform extremely well. By the end of 2012, CMS expects to more than triple its total data sample, and hence to probe further the nature of this new particle. If this particle is indeed the SM Higgs boson, its properties and implications for the standard model will be studied in detail. If it is not the SM Higgs boson, CMS will explore the nature of the new physics that it implies, which may include additional particles that are observable at the LHC. In either case, searches will also continue for other new particles or forces that can be observed in future runs of the LHC at higher beam energies and intensities.
More information
- This statement is available online at: http://cmsexperiment.web.cern.ch/news/observation-new-particle-mass-125-gev
- CMS public website: http://cern.ch/cms
- CMS Higgs Seminar at CERN (Prof. Joseph Incandela)
- CERN Press Release, 4 July 2012 [ Also available in French ]
- ATLAS Experiment Higgs search results
- ATLAS Higgs Seminar at CERN (Prof. Fabiola Gianotti)
- Full video of the seminar
- Full video of the press conference
- Photographs from the seminar and press conference
- Photographs from the webcast at ICHEP
Event images and animation of real CMS collisions
Images:
- Two photons i.e. γγ (8 TeV) event display
- ZZ to two electrons and two muons (8 TeV) event display
- All images from this statement (including plots)
Animations:
- Two photons i.e. γγ (8 TeV) event display animation: CDS | YouTube
- ZZ to two electrons and two muons (8 TeV) event display animation: CDS | YouTube
- ZZ to 4 muons (7 TeV) event display animation: CDS | YouTube
Short movies about the Higgs
- Animation of the Higgs mechanism [with subtitles] See also [without subtitles]
- What is the Higgs? by Don Lincoln
- Higgs boson: How do we search for it? by Don Lincoln
References
More about the Higgs at CMS
- CMS Physics Analysis summary papers about the Higgs
- About the Higgs boson
- Simple explanations of Higgs search terminology
- Search for the Standard Model Higgs boson in CMS
- All CMS Higgs plots and results
About CMS Physics
- All CMS Papers
- All CMS Physics Analysis Summaries
- All CMS results
- Images of real collisions in CMS
- Animations of real collisions in CMS
About CMS
More information: http://cern.ch/cms, or contact: cms.outreach@cern.ch. CMS is one of two general-purpose experiments at the LHC that have been built to search for new physics. It is designed to detect a wide range of particles and phenomena produced in the LHC's high-energy proton-proton and heavy-ion collisions and will help to answer questions such as: "What is the Universe really made of and what forces act within it?" and "What gives everything mass?" It will also measure the properties of well-known particles with unprecedented precision and be on the lookout for completely new, unpredicted phenomena. Such research not only increases our understanding of the way the Universe works, but may eventually spark new technologies that change the world in which we live as has often been true in the past. The conceptual design of the CMS experiment dates back to 1992. The construction of the gigantic detector (15 m diameter by nearly 29 m long with a weight of 14000 tonnes) took 16 years of effort from one of the largest international scientific collaborations ever assembled: 3275 physicists (including 1535 students) plus 790 engineers and technicians, from 179 institutions and research laboratories distributed in 41 countries all over the world.
Footnotes
[1] ICHEP is the 36th International Conference on High Energy Physics, Melbourne, Australia during 4-11 July, 2012. The results will be presented jointly: in person at CERN and by real-time video link to ICHEP. [2] The electron volt (eV) is a unit of energy. A GeV is 1,000,000,000 eV. In particle physics, where mass and energy are often interchanged, it is common to use eV/c2 as a unit of mass (from E = mc2, where c is the speed of light in vacuum). Even more common is to use a system of natural units with c set to 1 (hence, E = m), and use eV and GeV as units of mass. [3] The standard deviation describes the spread of a set of measurements around the mean value. It can be used to quantify the level of disagreement of a set of data from a given hypothesis. Physicists express standard deviations in units called “sigma”. The higher the number of sigma, the more incompatible the data are with the hypothesis. Typically, the more unexpected a discovery is, the greater the number of sigma physicists will require to be convinced. [4] Confidence level is a statistical measure of the percentage of test results that can be expected to be within a specified range. For example, a confidence level of 95% means that the result of an action will probably meet expectations 95% of the time. [5] http://news.stanford.edu/news/2004/july21/femtobarn-721.html [6] http://cmsexperiment.web.cern.ch/news/cms-search-standard-model-higgs-boson-lhc-data-2010-and-2011 [7] http://cmsexperiment.web.cern.ch/news/blinding-and-unblinding-analyses
Papers Timeline
Papers Timeline lucasPhysics
PhysicsParticle physicists study Nature at its most fundamental level by observing particle collisions. By understanding the properties of the various types of particles and the forces that govern them, we can learn about the origins of the Universe itself. Use the menu to explore recent results and publications from the CMS Collaboration.
The CMS Collaboration has a broad physics programme, ranging from measurements of the Standard Model and the recently discovered Higgs boson, to studies of heavy-ion collisions, to searches for new particles, phenomena, and even extra dimensions in the Universe.
Learn More About Scientific Results
Recipe for a Universe
Recipe for a Universe Anonymous (not verified)Take a massive explosion to create plenty of stardust and a raging heat. Simmer for an eternity in a background of cosmic microwaves. Let the ingredients congeal and leave to cool and serve cold with cultures of tiny organisms 13.7 billion years later.
To understand the basic ingredients and the ‘cooking conditions’ of the cosmos, from the beginning of time to the present day, particle physicists have to try and reverse-engineer the ‘dish’ of the Universe. Within the complex concoction, cryptic clues hide the instructions for the cosmic recipe.
Slowly simmer
Space, time, matter... everything originated in the Big Bang, an incommensurably huge explosion that happened 13.7 billion years ago. The Universe was then incredibly hot and dense but only a few moments after, as it started to cool down, the conditions were just right to give rise to the building blocks of matter – in particular, the quarks and electrons of which we are all made. A few millionths of a second later, quarks aggregated to produce protons and neutrons, which in turn were bundled into nuclei three minutes later.
Then, as the Universe continued to expand and cool, things began to happen more slowly. It took 380,000 years for the electrons to be trapped in orbits around nuclei, forming the first atoms. These were mainly helium and hydrogen, which are still by far the most abundant elements in the Universe.
Another 1.6 million years later, gravity began to take control as clouds of gas began to form stars and galaxies. Since then, the heavier atoms of which we are all made (such as carbon, oxygen, and iron) have been continuously ‘cooked’ in the hearts of the stars. They are stirred in with the rest of the Universe each time a star comes to a spectacular end as a supernova.
The mystery ingredient
So far so good, but there is one small detail left out: cosmological and astrophysical observations have now shown that all of the above accounts for only a tiny 4% of the entire Universe. In a way, it is not so much the visible things, such as planets and galaxies, that define the Universe, but rather the void around them!
Most of the Universe is made up of invisible substances known as 'dark matter' (26%) and 'dark energy' (70%). These do not emit electromagnetic radiation, and we detect them only through their gravitational effects. What they are and what role they played in the evolution of the Universe are mysteries, but within this darkness lie intriguing possibilities of hitherto undiscovered physics beyond the established Standard Model.
Story of the Universe
Story of the UniverseFrom the Big Bang to Today's Universe
t < 10-43 s : The Big Bang
The universe is considered to have expanded from a single point with an infinitely high energy density (infinite temperature). Is there a meaning to the question "What existed before the big bang?"
t ≈ 10-43 s, 1032 K (1019 GeV, 10-34m): Gravity “freezes” out All particle types (quarks, leptons, gauge bosons, and undiscovered particles e.g. Higgs, sparticles, gravitons) and their anti-particles are in thermal equilibrium, meaning they are created and annihilated at an equal rate. They coexist with radiation (photons). Through a phase transition, gravity "freezes" out and becomes distinct in its action from the weak, electromagnetic, and strong forces. At this time, the other three forces cannot be distinguished from one another in their action on quarks and leptons. This is the first instance of the breaking of symmetry amongst the forces.
Inflation ceases, expansion continues
Grand Unification breaks. Strong and electroweak
forces become distinguishable

t ≈ 10-35 s, 1027 K (1016 GeV, 10-32 m) : Inflation The rate of expansion increases exponentially for a short period of time. The universe doubles in size every 10-34 s. Inflation stops at around 10-32 s, by which time the universe has increased in size by a factor of 1050. This is equivalent to an object the size of a proton swelling to 1019 light years across!
The whole universe is estimated to have had a size of ~1023 m at the end of the period of inflation. The universe we can see today, however, was only 3 m in size after inflation. This solves the problems of ‘horizon’ (how is it possible for two opposing parts of the present universe to be at the same temperature when they cannot have interacted with each other before recombination?) and ‘flatness’ (density of matter is close to the critical density).
t ≈ 10-32 s : Strong forces freezes out Through another phase transition the strong force "freezes" out and a slight excess of matter over anti-matter develops. This excess, at a level of 1 part in a billion, is sufficient to give the presently observed dominance of matter over anti-matter. The temperature is too high for quarks to remain clumped to form neutrons or protons and so they exist in the form of a quark-gluon plasma. The LHC can study this by colliding together high-energy nuclei.
Electroweak force splits
t ≈ 10-10 s, 1015 K (100 GeV, 10-18 m) : Electromagnetic and Weak Forces separate The energy density corresponds to that at LEP. As the temperature falls, the weak force "freezes" out and all four forces become distinct in their actions. The antiquarks annihilate with the quarks, leaving a residual excess of matter. W and Z bosons decay. In general, unstable massive particles disappear when the temperture falls to a value at which photons from the black-body radiation do not have sufficient energy to create a particle-antiparticle pair.
Quarks combine to make protons and neutrons
t ≈ 10-4 s, 1013 K (1 GeV, 10-16 m) : Protons and Neutrons form The universe has grown to the size of our solar system. As the temperature drops, quark-antiquark annihilation stops and the remaining quarks combine to make protons and neutrons.
t = 1 s, 1010 K (1 MeV, 10-15 m) : Neutrinos decouple The neutrinos become inactive (essentially, they do not participate further in interactions). The electrons and positrons annihilate and are not recreated. An excess of electrons is left. The neutron-proton ratio shifts from 50:50 to 25:75.
Protons and neutrons combine to form helium nuclei
t = 3 minutes, 109 K (0.1 MeV, 10-12 m) : Nuclei are formed The temperature is low enough to allow nuclei to be formed. Conditions are similar to those that exist in stars today or in thermonuclear bombs. Heavier nuclei such as deuterium, helium, and lithium soak up the neutrons that are present. Any remaining neutrons decay with a time constant of ~ 1000 seconds. The neutron-proton ratio is now 13:87. The bulk constitution of the universe is now in place, consisting essentially of protons (75%) and helium nuclei. The temperature is still too high to form any atoms. Electrons form a gas of free particles.
The Universe becomes transparent and fills with light
t = 300 000 years, 6000 K (0.5 eV, 10-10 m) : Atoms are created Electrons begin to stick to nuclei. Atoms of hydrogen, helium, and lithium are created. Radiation is no longer energetic enough to break atoms. The universe becomes transparent. Matter density dominates. Astronomy can study the evolution of the Universe back to this time.
Galaxies begin to form
t = 109 years, 18 K : Galaxy Formation Local mass density fluctuations act as seeds for stellar and galaxy formation (the exact mechanism is still not understood). Nucleosynthesis, the synthesis of heavier nuclei such as carbon up to iron, starts occurring in the thermonuclear reactors that are stars. Heavier elements are synthesized and dispersed in the moments when stars collapse and supernova explosions occur.
Man begins to wonder where it all came from
t = 15 x 109 years, 3 K : Humans The present day. Chemical processes have linked atoms to form molecules. From the dust of stars and through coded messages (DNA) humans emerge and begin to observe the universe around them.
The Big Questions
The Big Questions Anonymous (not verified)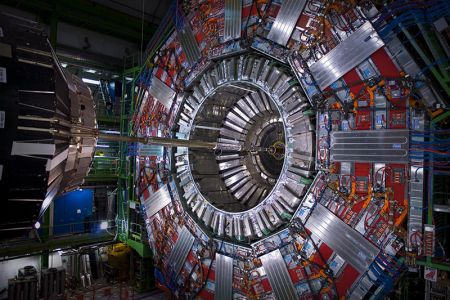
Accelerators and detectors
The particles that make up forces and matter are so small that if the nucleus of an atom were enlarged to the size of a tennis ball, a full stop (".") would be as big as the earth’s orbit of the sun. They are so infinitesimally small that no matter how hard physicists squint, they'll never see a quark with a microscope, let alone the naked eye. The key to studying the subatomic world is to use accelerators to boost the energy of particles before a collision, then make the results indirectly visible using detectors. But to appreciate why, we need to understand the incredible relationship between energy and mass.
Swapping energy for mass
Imagine you threw an unknown “mystery” ball at a target , and you wanted to know what type of ball it was. Knowing something about the target, the speed with which the ball bounces back and the track it makes when doing so, you might be able to deduce, for example, whether the ball is likely to be a small rubber ball or a heavy cannon ball. Using the same principle a particle accelerator throws particles with great speed and energy, while detectors provide information on the characteristics of the collisions. Physicists analyse the results of many collisions to gain an insight into the nature of the particles being studied, despite not being able to observe them directly.
To add to the challenge, in the rather bizarre world of particles, give the 'balls' enough of an energy boost and they may turn into something else entirely. That could be like shooting a ping-pong ball at a target to find it transforming into a truckload of watermelons and a handful of beads! This phenomenon is described by Einstein's famous equation E=mc2, which says that matter is a very concentrated form of energy and the two are interchangeable.
So when the Large Hadron Collider (LHC) collides simple protons at high energies, some of this energy converts into mass, creating exotic, heavy, short-lived particles. Particles like this were last common nearly 14 billion years ago, a tiny fraction of a second after the creation of the Universe at the Big Bang, and can open a window onto how our Universe was formed and how it is today.
How can accelerators and detectors “see” the subatomic world?
Our eyes can see objects because of visible light reflecting off their surfaces, but we can’t see particles with the naked eye because their sizes are smaller than one ‘unit’ (wavelength) of visible light.
Early in the 20th century it was discovered that moving particles of matter can also be considered as waves and that the wavelengths of these particles become shorter with increasing energy. This means that to study details a billion times smaller, we need to give particles energies a billion times higher. This forms the basic principle of how an accelerator can be used to measure the subatomic world.
Accelerating particles to high energies also allows us to see particles that would usually turn into other, more stable, particles too quickly for us to see them. This is due to another of Einstein’s great discoveries, Special Relativity, which tells us that when something travels fast, to stationary observers, its time appears to run slower. This effect is negligible at everyday speeds but for a particle travelling at close to the speed of light, time passes slow enough for the particle to travel much further than we would otherwise expect. This effect allows us to detect the beauty quark, which decays after just a picosecond (a trillionth of a second). At low speeds it decays quickly and doesn't travel through enough of the detector for us to see it, but when accelerated to near the speed of light, the particle covers a few extra millimetres: far enough for the detector to be able to spot it.
Sensing the subatomic is a job for giants
Something that may appear strange about particle physics experiments is the paradox between the sizes of the equipment and the subjects being investigated. Why does it take the largest machines to investigate something so unimaginably tiny?
The more energy you put into the particles, the more "stuff" (mass) can be created in a collision. And because the particles we are looking for are rare, the number of particles you collide must be high. As a result, studying particles that have never been investigated before often means building larger accelerators to reach higher energies and smashing particles together at higher rates. The history of particle physics may be traced back through a succession of accelerators and detectors of increasing sizes.
Similarly exciting collisions actually happen every day as high-energy particles from space hit particles in the Earth’s atmosphere, but the results go by, unobserved. What you need is a giant detector like CMS to analyse the results of the collisions. CMS’s huge size is dictated by the need to contain all the energetic particles produced in the collisions, and by the methods of identifying them.
Go to the "Detector" section to read more on why CMS is so big
Antimatter in CMS
Antimatter in CMS Anonymous (not verified)Spot the difference
So how did the universe evolve into this very asymmetric state, dominated by matter, if the underlying forces can barely tell the difference between the two? One possible explanation is that there exists yet another, undiscovered, force in nature that is not matter-antimatter symmetric, i.e. has different effects on matter and antimatter. Another, more popular explanation is that the weak interaction, through which such particles decay, can actually distinguish between matter and antimatter particles.
A clue to the answer into this question may be provided by the phenomenon of Charge-Parity (CP) violation, discovered over four decades ago. CP violation implies that there is a small difference in the rates at which certain particles decay and the corresponding rates at which their antiparticles decay.
One such particle is the B meson, a relative of the proton but made up of quarks known as “beauty”, “bottom” or more often just “b” quarks. Huge numbers of these will be generated in the LHC, and at CMS we can study their decay. Results from CMS will complement those generated in LHCb, a fellow LHC experiment that has been designed specifically for this task. Observing a large asymmetry in the decay rates of b quarks versus anti-b quarks might tell us more about why nature prefers matter over antimatter
The B meson and its antiparticle can both decay into two muons and a different meson made of “charm” quarks, which in turn decays further to form two pions (yet another of the meson family). This decay, shown in the diagram, presents a fairly simple signature for the experiment to detect. We can tell whether the decayed particle was the B meson or its antiparticle by looking at the type of muon produced by the decay of the opposite b quark in the event, and we will be able to compare the rates of the two.
Are there more Particles left to find?
Are there more Particles left to find? Anonymous (not verified)Supersymmetry: Uniting the forces
Towards a superforce
Our understanding of the workings of the Universe often progress when unexpected connections are found between what appeared at first to be separate entities. A major breakthrough occurred in the 1860s when James Clerk Maxwell recognized the similarities between electricity and magnetism and developed his theory of a single electromagnetic force. A similar breakthrough came a century later, when theorists began to develop links between electromagnetism, with its obvious effects in everyday life, and the weak force, which normally hides within the atomic nucleus. Vital support for these ideas came first from the Gargamelle experiment at CERN, and then with the Nobel prize winning discovery of the W and Z particles, which carry the electroweak force. But take note – it is only at the higher energies explored in particle collisions at CERN and other laboratories that the electromagnetic and weak forces begin to act on equal terms.
So will other forces join the club at even higher energies? Experiments already show that the effect of the strong force becomes weaker as energies increase. This is a good indication that at incredibly high energies, the strengths of the electromagnetic, weak and strong forces may be the same. The energies involved are at least a thousand million times greater than particle accelerators can reach, but such conditions would have existed in the very early Universe, almost immediately (10-34 s) after the Big Bang. Pushing the concept a step further, theorists even contemplate the possibility of including gravity at still higher energies, thereby unifying all the fundamental forces into a single force. This would have ruled the first instants of the Universe, before its different components separated out as the Universe cooled.
Enter superparticles
Although at present we cannot recreate conditions with energy high enough to test these ideas directly, we can look for the consequences of ‘grand unification’ at lower energies, for instance at the Large Hadron Collider. A very popular idea that allows the stong and electroweak forces to unite and become a single interaction at a common energy is called supersymmetry, or SUSY for short. SUSY provides a symmetry between matter and forces, and predicts that for each known particle there is a 'supersymmetric' partner. If this is correct, then supersymmetric particles should appear in collisions at the LHC.
Read more about Supersymmetry in CMS
Detecting Dark Matter
Detecting Dark Matter Anonymous (not verified)Evidence from the depths of the Universe has ruled out a number of models for what the mysterious dark matter might be, but one candidate that fits so far is the lightest supersymmetric particle (LSP) otherwise known as the “neutralino”, the lightest of a whole range of new particles suggested by a theory called supersymmetry. If the neutralino exists it will likely be stable, heavy, neutral, and will not interact electromagnetically. This makes it a perfect candidate for a substance that pervades the universe without being spotted.
If supersymmetric particles exist, they are very likely to be produced in collisions in the LHC. The heavy particles will decay into combinations of leptons (like electrons and muons) and quarks (which will cause sprays of particles called jets) as well as into neutralinos that will not decay any further. Therefore many neutralinos will pass through the CMS detector, without depositing any energy or leaving a trail.
So how do you detect an “invisible” particle? CMS will be able to find the neutralino indirectly – by identifying when the energy used to make it goes missing.
Momentum in equals momentum out…
One of the most fundamental laws of physics is that ‘momentum is conserved’. In other words, the total momentum before a collision is equal to the total momentum after. If the total momentum of the observed particles that emerge from a proton-proton collision does not equal the momentum of the two protons, we can deduce there must be an invisible particle somewhere that carried away that missing momentum.
As we collect all the particles we can also add up their momenta and energies (in the “transverse” direction, i.e. at right angles to the beam line) and reconstruct the entire collision; like building a giant jigsaw puzzle. When a neutralino is formed and we can’t detect it we see an imbalance in the collision, with particles flying out one side but not the other and the energy not adding up. This shows up as a hole in the jigsaw puzzle: a missing particle seen through its missing energy or momentum.
The CMS is “hermetic” when it comes to finding missing particles. This means that, to the extent possible, it catches every detectable particle emerging from a collision. Large detectors have “channels for escape”, regions where particles cannot be detected because of cables or other mechanical support. These regions must be minimised to ensure that standard particles can't slip by undetected. This way, if the energy or momentum is "missing", it really is due to an invisible particle.
To search for this missing energy, it is important that the CMS has a good hadron calorimeter as well as detectors at every angle around the beam line. To ensure that particles flying from all directions will be detected, this includes the very shallow angles known as the “forward region”.
Detecting extra dimensions
Detecting extra dimensions Anonymous (not verified)Superstring theory is elegant but speculative and throws up a number of possible experimental outcomes, some more likely than others.
One option would be to find evidence of another host of particles that can only exist if there are more dimensions. Theories that postulate these extra dimensions predict that, like an atom having a low energy ground state and then more energetic states, there must be heavier versions of standard particles recurring at higher and higher energies as they navigate smaller dimensions. These have been called Kaluza-Klein recurrences and would have exactly the same properties as standard particles (and so be visible to our detector) but at a greater mass. If CMS were to find a Z-like particle (the Z boson being one of the carriers of the electroweak force) at 2 TeV for instance, this might suggest the presence of extra dimensions. Such heavy particles wouldn't have been seen at lower energies.
As gravity is thought to be a force able to probe these extra dimensions, another way in which we may be find evidence for string theory is through the disappearance of gravitons, the hypothesised carrier of gravity, into these other dimensions. The particle might be carried away without a trace, but it would leave behind an imbalance in momentum and energy.
Two of the most fundamental laws of physics are that momentum and energy “are conserved”. In other words, the total momentum and energy before a collision is equal to the total momentum and energy after. So if we add these up in all the observed particles emerging from a collision (in the case of CMS we do this is the sideways “transverse” direction) and it does not equal the amount in the original particles, there must be a missing particle that carried away the momentum and energy: a lost piece leaving a hole in the jigsaw.
This is why the detector must be as “hermetic” as possible, that is, it must be able to catch, to the extent possible, every particle emerging from the collisions, so we can deduce that particles have genuinely disappeared, and have not just been missed by the detector.
This method of searching is similar to the way in which we will detect supersymmetric particles , so careful analysis would be needed to decide if a graviton has travelled to another dimension or we have instead produced a SUSY particle, whose decay products would also be invisible to our detector.
Finally, another spectacular yet speculative way of revealing extra dimensions would be through the production of “Micro Quantum Black Holes”, which, if there are extra dimensions, might be produced at the LHC. What exactly we would see would depend on the number of extra dimensions, the mass of the black hole, the size of the dimensions and the energy at which the black hole occurs. If micro black holes do appear they would disintegrate extremely rapidly, in around 10-27 seconds, as they decay into Standard Model or SUSY particles, producing many jets and leptons.
To find these would be an incredible feat that would tell us about how quantum mechanics, thermodynamics and gravity all work together on miniscule scale, allowing us to study quantum gravity in the laboratory. We could use what we see in our three dimensions - for instance, the type of particles emerging, their energy distributions and masses - to decipher the geometry of the extra dimensions and this would be a challenge for physicists to unravel for the coming century.
Do we really live in only three dimensions?
Do we really live in only three dimensions? Anonymous (not verified)String theory and extra dimensions
Loose ends
Will the string tie the Standard package? Hot on the heels of the Standard Model, some physicists are working to support an idea called string theory. This attempts to tie up the loose ends in the Standard Model by explaining all the fundamental particles and forces (including gravity) in a unified framework.
Underlying string theory is the radical idea that fundamental particles are not really like points or dots, but rather small loops of vibrating strings. All the different particles and forces are just different oscillation modes of a unique type of string. Bizarrely, the theory also implies that besides the familiar three–dimensional world and the fourth dimension of time, there are six additional spatial dimensions! These extra dimensions are apparently 'curled up' so small that we do not see them.
Which string?
String theory is conceptually complex, with a fascinating but very difficult mathematical structure. This has so far prevented researchers from deriving concrete predictions from the theory for comparison with experimental results. Not only does string theory involve the complex study of the geometry of extra dimensions, but the way the structure of the dimensions are chosen appears arbitrary and can lead to different outcomes.
For instance, there seem to be many possible ways to curl up the extra dimensions, by choosing different shapes and sizes. This leads to many alternative versions of the theory. In certain cases, the sizes of the extra dimensions are very small and it will be difficult to obtain direct evidence for them. In others, the sizes are far larger and could be observed at new accelerators such as CERN’s Large Hadron Collider.
Secret dimensions
In everyday life, we inhabit a space of three dimensions – a vast ‘cupboard’ with height, width and depth, well known for centuries. Less obviously, we can consider time as an additional, fourth dimension, as Einstein famously revealed. But just as we are becoming more used to the idea of four dimensions, some theorists have made predictions wilder than even Einstein had imagined.
String theory intriguingly suggests that six more dimensions exist, but are somehow hidden from our senses. They could be all around us, but curled up to be so tiny that we have never realized their existence.
What is a dimension?
Dimensions are really just the number of co-ordinates we need to describe things. We can compare this to a tightrope walker travelling along a rope. For the acrobat there is only one dimension – forwards or backwards, and we can state her or his position with just one number. But if we look on a smaller level, for an ant crawling about on the rope there would be two dimensions of travel: we’d need to know how far around the rope it is, as well as how far along. If we zoom in even further, for atoms inside the rope, the world would be in three dimensions, the x, y and z of everyday coordinates. Who is to say that as we go smaller and smaller the number of directions to travel in, the number of dimensions, does not increase even further?
Beyond the third dimension
Some string theorists have taken this idea further to explain a mystery of gravity that has perplexed physicists for some time – why is gravity so much weaker than the other fundamental forces? Why do we need objects the size of planets in order to feel its force when we can experience the electromagnetic force with just a small magnet? And if gravity’s quantum carrier, the graviton, exists, how can we find it? The idea is that we do not feel gravity’s full effect in the everyday world. Gravity may appear weak only because its force is being shared with other spatial dimensions.
To find out whether these ideas are just products of wild imaginations or an incredible leap in understanding will require experimental evidence. But how?
High-energy experiments could prise open the inconspicuous dimensions just enough to allow particles to move between the normal 3D world and other dimensions, manifesting itself in the sudden appearance or disappearance of a particle. Or we might detect some of the new phenomena that a world with extra dimensions predicts. Who knows where such a discovery could lead!
Read more about Extra dimensions in CMS via the link below
Heavy Ions in CMS
Heavy Ions in CMS Anonymous (not verified)Probing the Plasma
Lead nuclei consist of large numbers of protons and neutrons, both made up of quarks. When the nuclei collide, a range of particles will be produced, some of which are expected to behave differently if a QGP is produced and such behaviour will tell us something about the plasma.
The QGP will for instance affect the frequency with which we see special kinds of mesons made of a pair of “heavy” quarks. When no QGP is formed, quarks remain locked within their particles and a host of these particles fly into the detector, usually seen through their decays to muon pairs. However, when a QGP has been produced, the more loosely-bound of these mesons are no longer seen because they dissolve into the scalding quark soup.
When the plasma gets denser and hotter, the more strongly-bound of these particles also “melt”. By studying the number and types of the surviving particles we can then deduce something about the state of the matter and estimate the temperature of the QGP.
By looking at the energies of the detected jets (narrow sprays of particles produced by quarks or gluons) we can also deduce how dense the plasma is.
Jets are produced in pairs in collisions and fire out in opposite directions. Jets produced in the centre of the dense plasma are “quenched”, losing energy like bullets in water, and most never emerge. Those produced at the edge however might escape, but only in one direction, because the opposite jet will be lost to the plasma mass. If the plasma is dense enough we therefore expect to see single jets rather than jet pairs.
The barely emerging jet will have lost much of its energy. Knowing how much energy loss the plasma caused tells us how dense it is, but to calculate this loss we need to know the jet’s original energy. To do this we look at jets where on the opposite side of the collision a photon or Z boson was produced instead of the second jet. Because these particles do not interact via the strong force they can sail through the QGP unaffected, and tell us the original energy of the interaction.
Z bosons decay into two muons; therefore the final particles that CMS must detect are photons, muon pairs and jets. With very precise hadron and electromagnetic calorimeters and a high performance muon detector system, CMS is ideally equipped to probe the existence of the quark-gluon plasma.
How did Matter form?
How did Matter form? Anonymous (not verified)Colliding heavy nuclei
Clues to the early Universe
The Universe has changed a great deal in the 13.7 billion years since the Big Bang, but the basic building blocks of everything from microbes to galaxies were signed, sealed and delivered in the first few millionths of a second. This is when the fundamental quarks became locked up within the protons and neutrons that form atomic nuclei. And there they remain, stuck together with gluons, the carrier particles of the strong force. This force is so strong that experiments are not able to prise individual quarks or gluons out of their particles for long before they recombine quickly to produce new particles.
Primordial soup
Suppose, however, you could reverse the process. The current theory of the strong interaction predicts that at very high temperatures and very high densities, quarks and gluons should no longer be confined inside composite particles. Instead they should exist in a new state of matter known as ‘quark-gluon plasma’ (QGP).
Such a transition should occur when the temperature goes above a value around 2000 billion degrees - about 100 000 times hotter than the core of the Sun! For a few millionths of a second after the Big Bang the temperature of the Universe was indeed above this value; as it grew from being the size of a grapefruit right up to the size of our solar system, the entire Universe would have been in a state of quark-gluon plasma – a hot, dense ‘soup’ of quarks and gluons. Then as the Universe cooled below the critical value, the soup condensed into composite particles, including protons and neutrons.
Experiments at CERN’s Super Proton Synchrotron reported tantalising evidence for quark-gluon plasma in 2000 and the Relativistic heavy Ion Collider (RHIC) at Brookhaven National Laboratory has since pursued a broad and successful programme in which some fascinating and unexpected properties of the quark-gluon system where observed, giving us new insights into what to look for. The next big step will be with the Large Hadron Collider (LHC) .
For a period of time each year the LHC will, in place of colliding protons, collide heavy lead nuclei at close to the speed of light, recreating conditions similar to those just after the “Big Bang”, only on a much smaller scale. The quarks and gluons previously confined in each proton or neutron will then form part of a QGP. This will very quickly expand and cool and at a low enough temperature reassemble as ordinary matter. The reassembled particles fly out into the detector and studying them will help us understand how quarks and gluons behave in their plasma state. This in turn will help us to understand why and how these basic constituents of matter ever formed into protons and neutrons at all, and what keeps them in that state.
Read more about Heavy ions in CMS via the link below
Seeking Susy
Seeking Susy Anonymous (not verified)Will SUSY be found?
If supersymmetry exists it will likely show itself through the presence of missing energy and through some particle signatures being produced more frequently than the normal “background” rate. A thorough understanding of the detector and all possible read-outs is therefore necessary to establish that we can’t explain these results by any known phenomena or experimental error before looking to a new explanation.
CMS is well equipped to discover such elusive particles and if SUSY is a true symmetry of Nature at the energy scales of the LHC, it will almost certainly be discovered in CMS.
This means a doubling of every particle we know to make SUSY partners or “sparticles”: matter partners are given an “s”, so the partners of electrons and quarks are called “selectrons” and “squarks”, and force partners become an “ino”, for example a “gluino” for the gluon and a “zino” for the Z boson.

Given that in the 1930s physicist Paul Dirac already doubled the number of known particles by suggesting that each had an associated “antiparticle”, this idea isn’t so strange. But why haven't we ever seen these SUSY particles?
Physicists think that we’ve yet to see the superpartners because SUSY is a broken symmetry, where sparticles have much heavier masses and are less stable than their counterparts. So though SUSY particles would have featured in the same numbers as standard particles in the early Universe, as it expanded and cooled they would have decayed to now only exist in a lightest “fossil” form that is hard to find and detect. But if SUSY particles exist at energy scales compatible with the LHC, up to 2-3 TeV, we will be able to produce and study them in large quantities right here.
Does a SUSY have a dark side?
Because it is both heavy and undetectable, the LSP (neutralino) is a good candidate for dark matter. This relies on the particle being perfectly stable, since dark matter has to have survived from the Big Bang until now. If the LSP is not stable, we can still detect it in CMS through its decay to other particles, rather than through missing energy, but dark matter will remain a mystery.
How to detect a superparticle
SUSY particles produced in the LHC are likely to be pairs of gluinos and/or squarks, heavy particles that will only live for 10-23 seconds before they decay (turn into other more stable and familiar particles) in multiple stages, forming long “decay chains”. The final products will consist of leptons like electrons and muons (producing clean tracks in the experiment), quarks (producing sprays of particles known as jets), and always the lightest supersymmetric particle (LSP).

The LSP is thought to be the lightest of the four "neutralinos", formed as combinations of a zino, a photino and two higgsinos: the SUSY partners of the Z boson, photon and Higgs boson respectively. The LSP is thought to be stable and so marks the end of the decay chain. But because it is weakly interacting and neutral, like the neutrino, it will pass straight through material and not be detected in CMS.
So how do you detect a particle you can’t see and that doesn’t interact with your detector? CMS will be able to detect LSPs, and so SUSY particles, by instead spotting when energy or momentum from the initial collision goes missing.
Momentum in equals momentum out…
One of the most fundamental laws of physics is that ‘momentum is conserved’. In other words, the total momentum before a collision is equal to the total momentum after; in this instance zero, because the protons travel with equal speeds in opposite directions so their momenta cancel out. If the total momentum of the observed particles that emerge from a proton-proton collision does not equal zero, we can deduce there must be an invisible particle somewhere that carried away that missing momentum.
CMS does exactly this: collecting and adding up the momenta and energies of all the emerging particles from a collision (in the “transverse” direction, i.e. at right angles to the beam line). CMS reconstructs the collision like a giant jigaw, and if the emerging particles seem imbalanced, flying out one side but not the other because an undetected LSP has been produced, we see the missing particle as a hole in the puzzle, identifiable due to its missing momentum or energy.
To spot the missing particles CMS is “hermetic” meaning that, to the extent possible, it catches every detectable particle emerging from a collision. Large detectors have cables and support regions that don't detect any kinds of particle and these channels for escape must be minimised to ensure that standard particles can't slip by undetected. This way if the energy or momentum is ‘missing’ it really is due to an invisible particle.
Finding missing energy is why CMS needs a good hadron calorimeter and why it has detectors in the “forward region”, at very shallow angles to the beam line (in addition to the detectors that surround the collision at larger angles) so that particles flying in all directions will be picked up.
Another important consideration in detecting SUSY particles is the ability to detect the “beauty” or “bottom” quarks. The b quark features in a number of decay chains, its sparticle expected to be one of the lighter squarks, but only lives for a very short amount of time before decaying (1 picosecond, one millionth of a millionth of a second). To spot these detectors must have very fine resolution and be placed very close to the beam line.
SUSY signatures
Pairs of SUSY particles decay in multiple stages producing a cascade of particles, often including further SUSYs, but resulting in three main “signatures” that CMS can look for.

The first uses the fact that the final decay products will often consist of pairs of leptons of opposite charge (e.g. a muon and an anti-muon), along with the ever present LSPs. Looking for unusually high levels of pairs of these particles should be a clear signal of SUSY production and is why we need a high performance detector system for muons , and a good ECAL to detect electrons.
The second common SUSY decay is for gluino or squark pairs to decay into lots of quarks and anti-quarks, along with the two LSPs; in the experiment seen as many narrow sprays of particles called jets and missing energy.
The third way involves spotting an otherwise rare occurrence, the production of pairs of leptons with the same charge. Usually pairs of leptons are produced from decays of a pair of oppositely charged particles, or from the decay of a single neutral particle; either way, conservation of charge means that the two leptons will have opposite charges. However with SUSY we expect to produce pairs of gluinos, which are neutral particles. Furthermore the decay of each gluino is just as likely to produce a positively charged lepton as a negatively charged one (balanced by negatively or positively charged quarks), so a pair of gluinos will often give a pair of leptons with the same charges. Finding unexpectedly large number of same sign leptons produced in events (e.g. two muons rather than a muon and an anti-muon) would also strongly point towards SUSY production.
SUSY Higgs

Supersymmetry doesn’t only increase the number of familiar particles: within supersymmetric models, there are also at least five Higgs bosons – the light scalar (ho), the heavy scalar (Ho), the pseudo scalar (Ao) and the negative and positive charged Higgs particles (H±). These can decay to photons, leptons (including the heaviest known lepton, the tau) and mesons (particles made of quarks), seen as jets in the detector.
Shown in the decay diagram and event display is how a SUSY Higgs might decay to two taus, giving an end product of an electron, jets (caused by the pions) and neutrinos, which will be detected through their missing energy. If supersymmetric Higgs particles exist, one way they could therefore be seen is as an increased frequency of pairs of taus produced as specific energies, as shown in the graph.

What and where is Antimatter?
What and where is Antimatter? Anonymous (not verified)Antimatter detectives
The antimatter is missing – not from CERN, but from the Universe! At least that is what we can deduce so far from careful examination of the evidence. For each basic particle of matter, there exists an antiparticle with the same mass, but the opposite electric charge. The negatively charged electron, for example, has a positively charged antiparticle called the positron. When a particle and its antiparticle come together, they both disappear, quite literally in a flash, as the annihilation process transforms their mass into energy.
The evidence spoke for itself
The ‘case file’ of antimatter was opened in 1928 by physicist Paul Dirac. He developed a theory that combined quantum mechanics and Einstein’s special relativity to provide a more complete description of electron interactions. The basic equation he derived turned out to have two solutions, one for the electron and one that seemed to describe something with positive charge (in fact, it was the positron). Then in 1932 the evidence was found to prove these ideas correct, when the positron was discovered occurring naturally in cosmic rays.
For the past 50 years and more, laboratories like CERN have routinely produced antiparticles, and in 1995 CERN became the first laboratory to create anti-atoms artificially. But no one has ever produced antimatter without also obtaining the corresponding matter particles. The scenario should have been the same during the birth of the Universe, when equal amounts of matter and antimatter would have been produced in the Big Bang.
“Just one more thing…”
So if matter and antimatter annihilate, and we and everything else are made of matter, why do we still exist? This mystery arises because we find ourselves living in a Universe made exclusively of matter. Didn't matter and antimatter completely annihilate at the time of the Big Bang? Perhaps this antimatter still exists somewhere else? Otherwise where did it go and what happened to it in the first place?
Such questions have led to speculative theories, from a break in the rules to the existence of an entire anti-Universe somewhere else! The way to solve the baffling disappearance of antimatter, and to learn more about this substance in general, is by studying both particles and antiparticles to find and decipher the subtle clues.
Click the link below to read more about Antimatter in CMS
What do we already know?
What do we already know? Anonymous (not verified)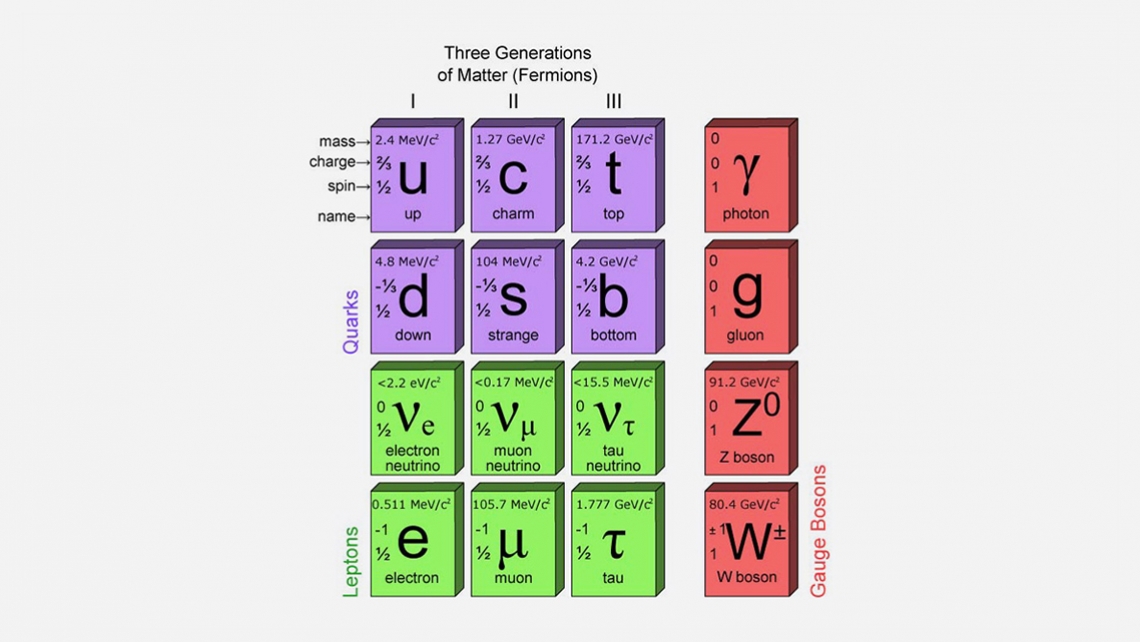
The standard package
The theories and discoveries of thousands of physicists over the past century have resulted in a remarkable insight into the fundamental structure of matter: everything that has been directly observed in the Universe until now has been found to be made from twelve basic building blocks called fundamental particles, governed by four fundamental forces. Our best understanding of how these twelve particles and three of the forces are related to each other is encapsulated in the Standard Model of particles and forces. Developed in the early 1970s, it has successfully explained a host of experimental results and precisely predicted a wide variety of phenomena. Over time and through many experiments by many physicists, the Standard Model has become established as a well-tested physics theory.
Matter particles
Everything around us is made of matter particles.These occur in two basic types called quarks and leptons.
Each group consists of six particles, which are related in pairs, or ‘generations’. The six quarks are paired in the three generations – the 'up quark' and the 'down quark' form the first generation, followed by the 'charm quark' and 'strange quark', then the 'top quark' and 'bottom quark'. The six leptons are similarly arranged in three generations – the 'electron' and the 'electron-neutrino', the 'muon' and the 'muon-neutrino', and the 'tau' and the 'tau-neutrino'. The electron, the muon and the tau all have an electric charge and mass, whereas the neutrinos are electrically neutral with very little mass.
The lightest and most stable charged particles are in the first generation, whereas the heavier and less stable particles belong to the second and third generations. All stable matter observed in the Universe is made from particles that belong to the first generation; any heavier particles quickly decay to the next most stable level.
Forces and carrier particles
There are four fundamental forces at work in the Universe: the strong force, the weak force, the electromagnetic force, and the gravitational force. They work over different ranges and have different strengths. Gravity is the weakest but it has an infinite range. The electromagnetic force also has infinite range but it is many times stronger than gravity. The weak and strong forces are effective only over a very short range (the size of a proton) and dominate only at the level of subatomic particles. Despite its name, the weak force is much stronger than gravity but it is indeed the weakest of the other three. The strong force is, as the name implies, the strongest among all the four fundamental interactions.
We know that three of the fundamental forces result from the exchange of force carrier particles, which belong to a broader group called ‘bosons’. Matter particles transfer discrete amounts of energy by exchanging bosons with each other. Each fundamental force has its own corresponding boson particle – the strong force is carried by the ‘gluon’, the electromagnetic force is carried by the ‘photon’, and the ‘W and Z bosons’ are responsible for the weak force. Although not yet found, the ‘graviton’ should be the corresponding force-carrying particle of gravity.
The Standard Model includes the electromagnetic, strong and weak forces and all their carrier particles, and explains extremely well how these forces act on all the matter particles. However, the most familiar force in our everyday lives, gravity, is not part of the Standard Model. In fact, fitting gravity comfortably into the framework has proved to be a difficult challenge. The quantum theory used to describe the micro world, and the general theory of relativity used to describe the macro world, are like two children who refuse to play nicely together. No one has managed to make the two mathematically compatible in the context of the Standard Model. But luckily for particle physics, the effects of gravity on their experiments have been so weak as to be negligible. Only when we have matter in bulk, such as in ourselves or in planets, is gravity observed to dominate. So the Standard Model still works well despite its reluctant exclusion of one of the fundamental forces.
So far so good, but...
...it is not time for physicists to call it a day just yet. Even though the Standard Model is currently the best description we have of the subatomic world, it does not explain the complete picture. The theory incorporates only three out of the four fundamental forces, omitting gravity. Alas, Newton would be turning in his grave! Nor does it explain why the many well-established basic parameters such as particles' masses have the values they do. There are also important questions it cannot answer, such as what is dark matter, what happened to the missing antimatter, and more.
Last but not least, an essential ingredient of the Standard Model, a particle called the Higgs boson, has yet to be found in an experiment. The race is on to hunt for the Higgs – the key to the origin of particle mass. Finding it would be a big step for particle physics, although its discovery would not write the final ending to the story.
So despite the Standard Model's effectiveness at describing the phenomena within its domain, it is nevertheless incomplete. Perhaps it is only a part of a bigger picture that includes new physics that has so far been hidden deep in the subatomic world or in the dark recesses of the Universe. New information from experiments at the Large Hadron Collider are sure to help us find more of these missing pieces.
What is the Universe really made of?
What is the Universe really made of? Anonymous (not verified)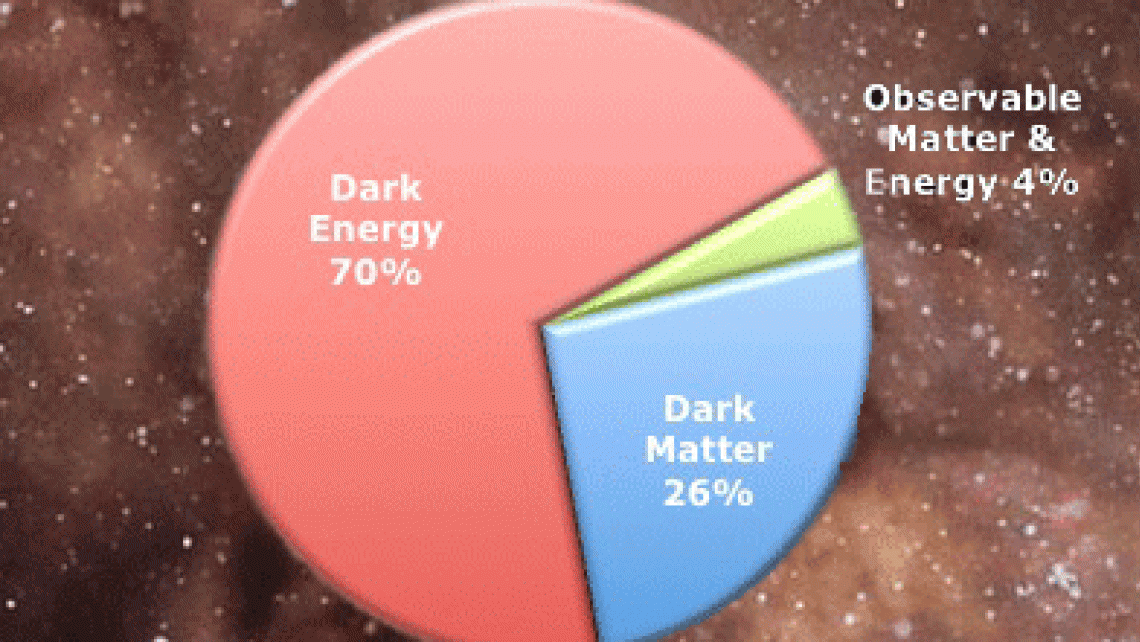
Dark secrets of the Universe
It’s perhaps natural that we don’t know much about how the Universe was created – after all, we were never there ourselves. But it’s surprising to realise that when it comes to the Universe today, we don’t necessarily have a much better knowledge of what is out there. In fact, astronomers and physicists have found that all we see in the Universe – planets, stars, galaxies – accounts for only a tiny 4% of it! In a way, it is not so much the visible things that define the Universe, but rather the void around them.
Cosmological and astrophysical observations indicate that most of the Universe is made up of invisible substances that do not emit electromagnetic radiation – that is, we cannot detect them directly through telescopes or similar instruments. We detect them only through their gravitational effects, which makes them very difficult to study. These mysterious substances are known as ‘dark matter’ and ‘dark energy’. What they are and what role they played in the evolution of the Universe are a mystery, but within this darkness lie intriguing possibilities of hitherto undiscovered physics beyond the established Standard Model.
Dark matter
Dark matter makes up about 26% of the Universe. The first hint of its existence came in 1933, when astronomical observations and calculations of gravitational effects revealed that there must be more 'stuff' present in the Universe than telescopes could see.
Researchers now believe that the gravitational effect of dark matter makes galaxies spin faster than expected, and that its gravitational field bends the light coming from objects behind it. Measurements of these effects show that dark matter exists, and they can be used to estimate the density of dark matter even though we cannot directly observe it.
But what is dark matter? One idea is that it could contain ‘supersymmetric particles’ - hypothesized particles that are partners to those already known in the Standard Model. Experiments at the Large Hadron Collider may be able to find them.
Dark energy
Dark energy makes up approximately 70% of the Universe and appears to be associated with the vacuum in space. The LHC will not directly detect dark energy.
The Size of Things
The Size of ThingsWhy do physicists need enormous machines, like the Large Hadron Collider (LHC) and gigantic viewing instruments (detectors) such as the Compact Muon Solenoid (CMS) to conduct their investgations of the sub-atomic world?
The size and position of an object tends to dictate what type of instrument is required to observe it. For example, what instruments would be helpful for the following backyard-observations: 1) a tree in your garden, 2) a small bird sitting on the highest branch of the tree, 3) the moon in the sky above the tree ? Your eye, a pair of binoculars, and a telescope would assist in the study of each of these items, respectively.
Now… there is an odd [inverse] relationship when people want to study very small objects : The smaller the object to be viewed, the larger the viewing instrument is. For example, to examine an ant, a virus, or an atom, a person would correspondingly need an optical microscope, an electron microscope, or a scanning tunneling microscope. To go further than this requires a particle accelerator, the first of which used energetic electron beams.
The following was written by Prof. Leon Lederman, winner of the Nobel Prize in Physics, and taken from the foreward of Don Lincoln's excellent book about the LHC: "The Quantum Frontier":
"These were invented in the 1930s and provided precise data on the size, shape and structure of atoms. To probe the nucleus of atoms, higher energies were required, and the acceleration of protons was added to the toolkit of physicists.
An approximate timetable of progress in accelerators may be useful and is shown below. Note that eV equals one electron volt, so keV is 103 electron volts and so on. You can see in the table that the higher the energy of the accelerated particle, the smaller the distance probed. However, to probe the very small, the accelerators also grew in size, complexity and cost. Accelerators are then in essence powerful microscopes, taking over when light is no longer sufficient."
Date | Energy | Distance Probed |
---|---|---|
1930
|
~100 keV
|
10-11 metres
|
1950
|
~100 MeV
|
10-14 metres
|
1970
|
~100 GeV
|
10-17 metres
|
1990
|
1 TeV
|
10-18 metres
|
2010
|
~10 TeV
|
10-19 metres
|
2030
|
?
|
?
|
Publishing an Analysis
Publishing an AnalysisPhysics collaborations such as those at large particle accelerator facilities at CERN or Fermilab are organic entities in themselves! Hundreds of minds gather together with the aim of producing results that the world has not seen before. Different cultures, different backgrounds and even different approaches help achieve the common goals in everyone’s sights.
It is important to understand how such a large group of people present their physics results to the rest of the community: A scientific paper written by a single person is the product of one mind; a paper by a collaboration the size of CMS is the product of over 2000 physicists.
Each paper goes through an exhaustive journey from early analysis to publication. This journey has several fixed waypoints, which exist so that every CMS member (who will sign the paper eventually) has the possibility to look at it. Everybody in the collaboration has the right and duty to contribute to the paper and must duly be informed when a paper is in production.
Beginning an analysis
As data for analysis starts to be collected from the LHC’s collisions, the life of a CMS paper typically begins with one of the many physics working groups such as those dedicated to studies of the Top quark, the Higgs boson, Supersymmetry, and so on. Each of these working groups has conveners who co-ordinate the analyses made by its members.
Physicists wishing to perform a particular analysis approach the conveners of the respective working group with a proposal in the form of a presentation. The conveners then decide whether to proceed with the analysis or not. If they decide to go ahead, the analysis topic gets added to the CMS Analysis Database and can be accessed via its Interface (CADI).
Any member of the collaboration can access CADI to find a list of the topics currently being analysed along with a list of authors responsible for them. The topics under analysis are not a mystery; it is known who the participants are, it is well known where more effort is needed and people are welcome to join.
Pre-approval
The authors regularly present their studies at working group meetings and as the analysis makes progress it proceeds to the stage known as the pre-approval. The analysis must be ready for all members of the collaboration to see at least one week before the pre-approval meeting. The last leg before this pre-approval involves the Analysis Review Committee (or ARC), which is typically made up of three people appointed by the Physics Co-ordinator and the Publications Committee Chairperson.
Appointing a committee is not easy. The large collaboration has people with different skills and it is important that the right people be selected for the committees. Senior members are often asked to nominate others for this role.
The conveners decide to hold the pre-approval meeting when they are satisfied with the progress the authors have made. At this meeting, one of the authors presents their findings to other members of the collaboration.
Discussions during or after the presentation can sometimes get very heated. Yes, everyone might belong to the same team, but no punches are pulled when it comes to passing a critical eye over the work of one’s colleagues; perhaps this is so because everyone expects the papers to which they are signatories to be watertight. It is healthy that different people have different points of view.
Approval talk
While the working group is responsible for oversight of the analysis before pre-approval, after this crucial point the ARC assumes responsibility. When the authors have answered all the questions raised at the pre-approval and during the ARC review, and have made any necessary modifications, the analysis must be approved by the collaboration. The approval talk is announced two weeks before it is to occur. Preferably any discussion should happen before the meeting, not at the last moment. So, the documentation for the analysis is frozen and made available at the same time as the announcement so collaboration members can read it.
Documentation is important, including that which is only circulated internally. Ideally, other physicists should be able to reproduce the analysis themselves after reading the paper. Not everyone in the collaboration will look at all of it, but the level of scrutiny is sufficient that no paper produced would be rejected by a journal.
At the approval talk, CMS members ask questions after the presentation. The Physics Co-ordinator listens to questions and replies, and tries to understand if there are important points that need more scrutiny. The ARC then gives its opinion — to approve the analysis or do more work. After approval of the physics, if the paper as a whole is not ready but CMS would like to present analysis to a conference, a Physics Analysis Summary (PAS) is made available for distribution outside the collaboration.
Collaboration-wide review
There is another important stage before the paper is ready to be published. The draft of the paper is circulated for a collaboration-wide review (CWR) — which could happen in parallel with the analysis approval process or after it. In the CWR, signatories to the paper comment on text, and on the physics described in the text.
There is a distinction between these two, sometimes parallel, processes: While the physics approval is for CMS as a whole, the CWR includes all people who sign the paper — a set that overlaps with CMS members but includes others as well. There are a few people that sign the paper but who are no longer members of the CMS collaboration. When you join CMS, you start signing the papers one year after you have joined and you stop signing one year after you have left the collaboration. There are rules for people who have collaborated with CMS in the past, because work on the experiment started over 15 years ago.
Final Reading
After an analysis has been approved and the paper passes the CWR, the ARC, authors and others go over the entire paper in what is known as the Final Reading. This is the final stage of the paper before it leaves the collaboration and is done to ensure that all CMS papers communicate physics with clarity, have the same style and retain the same form. At the Final Reading, the paper is “blessed” by the Publication Committee, after which it is frozen and sent to a journal, where the CMS paper goes through an anonymous peer-review process, as would papers from smaller collaborations or individual scientists. Sometimes, there are suggestions from the referees although most papers are published without major changes.
- This text is a shorter version of an article that appreared in the CMS Times on 21st February, 2011, under the name Life of a CMS Paper.